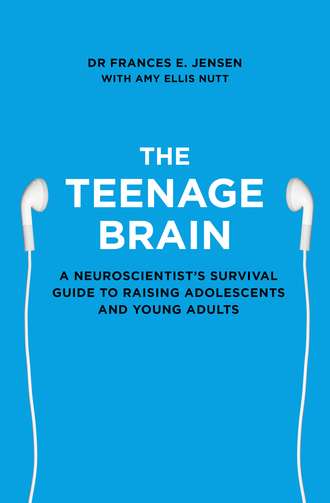
Полная версия
The Teenage Brain: A neuroscientist’s survival guide to raising adolescents and young adults
If you think of each neuron’s cell body as a point in a relay, there must be an incoming and an outgoing signal. Once the outgoing signal reaches the axonal bouton, or end point, it sets off a reaction causing the bouton to release packages of chemical messengers, called neurotransmitters. The point of contact between two neurons is called a synapse, and is actually a space no more than two-millionths of an inch wide. The synapse is truly where the action takes place in the brain. The signal heads down the neuron through the axon to the synapse and is then released as a chemical message. Like liquid keys, these neurotransmitters cross the synapse and lock onto the neuron on the other side, and in this way carry information from one cell to another. Once opened, the receptor causes a chain reaction of signals going down the receiving cell, triggering a pulse, or an action potential, which travels from a dendrite and through the cell body and out the axon toward another cell.
In order for neurons to survive, they need helper cells called glia. There are several types of glia: astrocytes, microglia, and oligodendrocytes. To put it simply, the astrocytes defend the neuron by helping to nourish it and cleaning up the unwanted chemicals around it. This helps keep the brain’s neurons at optimal functioning level. The microglia are tiny cells that move around the neuron and really activate when there is an infection or inflammation—they move through brain tissue to the site of action to fight these injuries, like an army-in-waiting. But because the brain is efficiently designed, microglia also have an everyday purpose, a kind of housekeeping duty, so that even when they are not activated, they are still helping maintain the health and well-being of the synapses. Oligodendrocytes are the cells that make the myelin that goes around the axon of neurons. These cells are tightly packed in the white matter, wrapping whitish-colored myelin around axons to insulate them, much like rubber around an electrical cord, allowing faster speeds of signal transmission down the axon.
While you are born with the vast majority of your neurons, most of the synapses in the cortex are not fully formed. In lower areas, like the brainstem, synapses are indeed almost fully mature. In the cortex, however, synapses are produced after birth in a burst of activity, which I mentioned earlier, known as the critical period. During this stage of development, a baby’s brain creates an astonishing two million synapses every second, allowing the infant to reach mental milestones like color vision, grasping, facial recognition, and parental attachment. It’s as if an infant’s brain is sending out billions of antennae, scanning the world for information. For each synapse to survive, it must find another neuron to send information to; this is why the number of synapses in a baby’s brain peaks in childhood. The gray matter—the brain tissue responsible for processing information—continues to thicken throughout childhood as the brain’s cells form extra connections, those limb-like dendrites. Known as arborization, this thickening is like a tree growing extra branches and roots. Stimulation, experiences, repeated sensations—all contribute to the creation of these new neural pathways. In adolescence, this “overgrowth” is responsible for a teen’s heightened capacity to learn new things quickly—everything from operating the new TV remote to speaking Mandarin Chinese. The profusion of gray matter, though, can also cause a kind of cognitive dissonance in which the brain has trouble picking out the right signals from all the “noise.” As a result, by late adolescence the brain has begun to prune away excess synapses and streamline connections.
Synapses come in two flavors: ones that excite, or turn on, the next neuron, and ones that inhibit, or turn off, the next neuron.
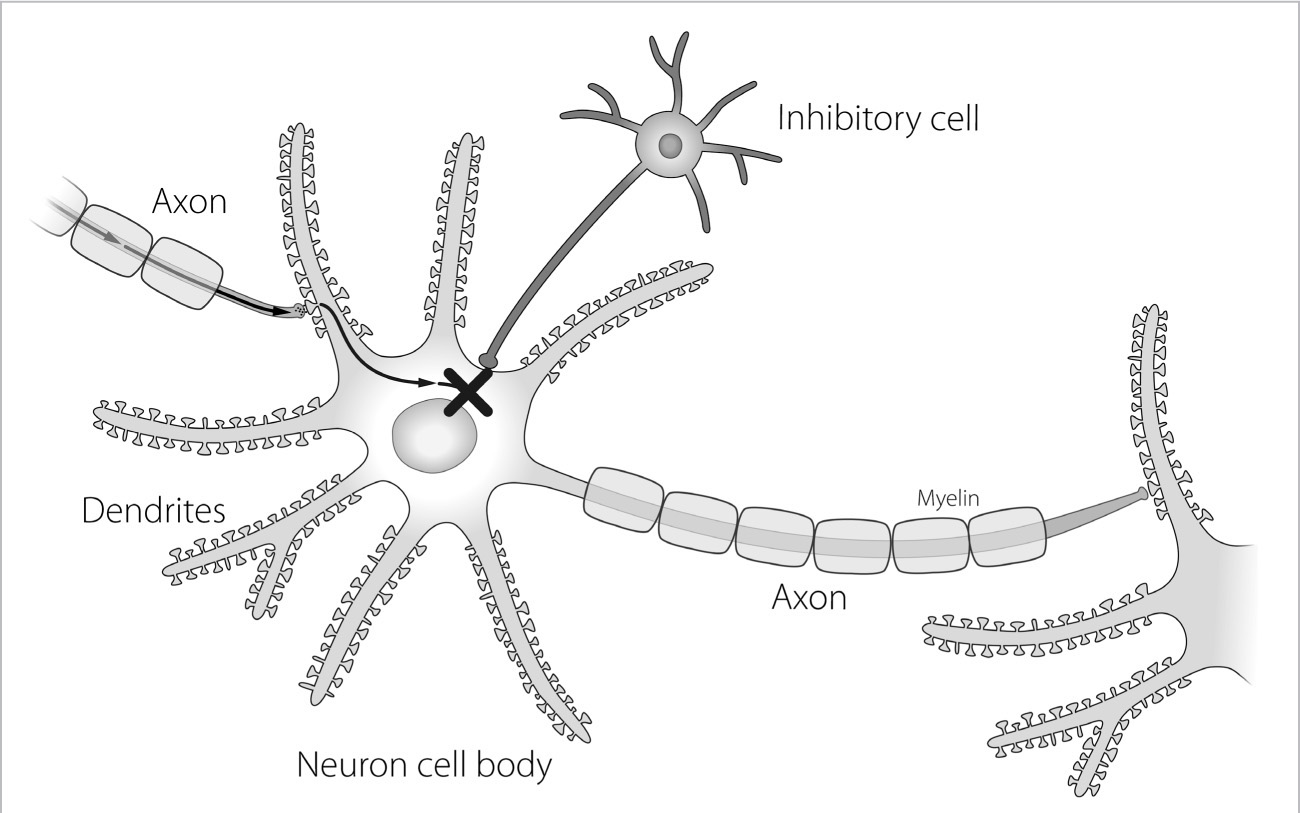
FIGURE 7A. Inhibitory Cells Can Stop Signaling: Inhibitory cells release inhibitory neurotransmitters onto spines, which will stop a signal in a neuron and turn the cell “off.”
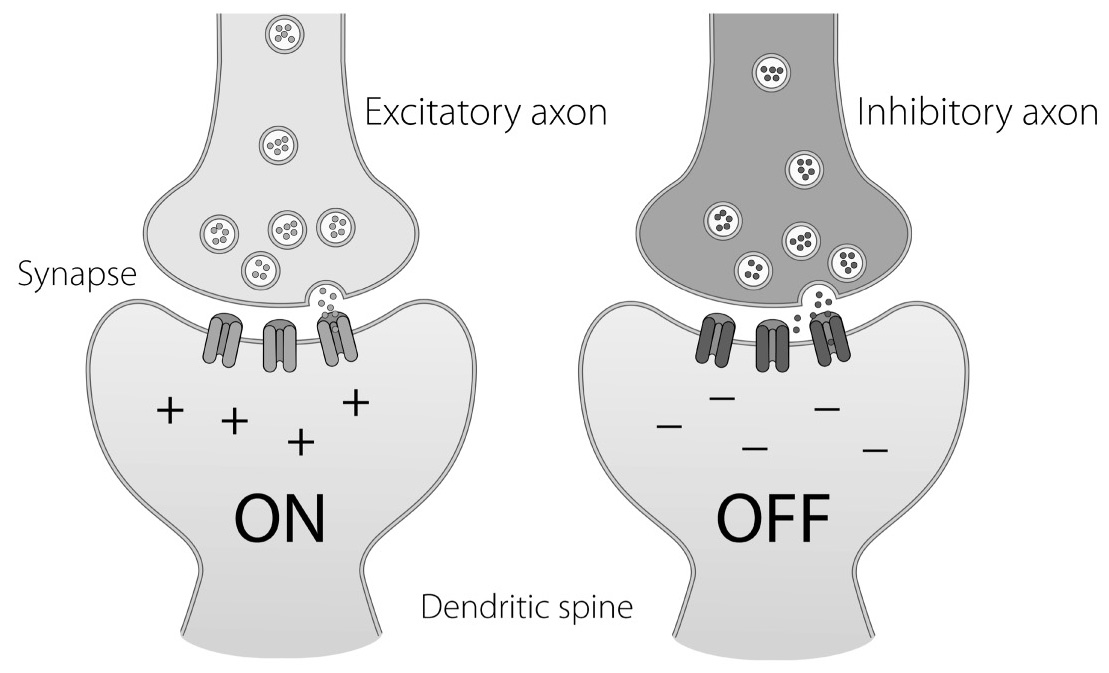
FIGURE 7B. Excitatory and Inhibitory Synapses: Excitatory axons release excitatory neurotransmitters, such as glutamate, which bind to excitatory receptors and turn the neuron “on.” Inhibitory axons release inhibitory neurotransmitters, like GABA, which bind to inhibitory receptors and turn the neuron “off.”
Whether or not the synapse is excitatory or inhibitory depends upon the type of neurotransmitter the axon puts out and also on the custom-made receptor, or lock, which is the part of the synapse poised to “receive” the neurotransmitter. If you imagine the neurotransmitter as a simple geometric shape, say a square or a circle, the specific receptor for that “flavor” of neurotransmitter will have the complementary shape in order to make a perfect fit. Just as “you can’t put a square peg in a round hole,” these neurotransmitter “keys” will fit into only the perfect receptor “locks.” This helps the synapse not confuse messages. In addition to the near-perfect pairing of neurotransmitters to receptors, another way the signal is kept clean is that the astrocyte helper cells immediately clean up any leftover neurotransmitter hanging around after it gets released. This happens in milliseconds, as the timing of these signals between brain cells has to be rapid, sharp as a burst.
Once the neurotransmitter has bound and locked itself into the receptor on the receiving neuron, this pairing sets off a chain reaction. Inside the dendritic side of the synapse, there are lots of proteins that rush to work when the synapse gets excited or inhibited. The signal needs to get down the dendrite to the cell body of the neuron, where it sends a positive charge for an excitatory signal or a negative charge for an inhibitory signal. Depending on which charge is sent, the receiving neuron will get a message to either stop or start functioning. If the message is positive, the receiving neuron will send the information down its own axon and across another synaptic cleft, and so on. A neuron can have up to ten thousand synapses and can send a thousand electrical impulses every second. In one-tenth of the time it takes to blink your eyes, a single neuron can simultaneously send a signal to hundreds of thousands of other neurons.
Some of the most common excitatory neurotransmitters are epinephrine, norepinephrine, and glutamate. Inhibitory neurotransmitters, like gamma-aminobutyric acid (GABA) and serotonin, act as antianxiety nutrients, calming the body and telling it to slow down. A lack of serotonin can result in aggression and depression. Dopamine is a special neurotransmitter because it is both excitatory and inhibitory. It is also, along with epinephrine and several others, a hormone. When it acts on the adrenal glands, it is acting hormonally; when it acts in the brain, it is a neurotransmitter. As a brain chemical messenger, dopamine helps motivate, drive, and focus the brain because it is integral to the brain’s reward circuitry. It’s the “I gotta have it” neurochemical that not only reinforces goal-directed activity but also can, in certain circumstances, lead to addiction. The more dopamine that is released in the brain, the more the reward circuits are activated, and the more those circuits are activated, the bigger the craving. It doesn’t matter if the craving is at the dinner table or the card table, in the boardroom or the bedroom. For instance, scientists know that high-calorie foods produce more dopamine in the brain. Why? Because higher calories increase our chance for survival. When we crave ice cream or gambling or sex, we may not actually be craving sweets, money, or orgasms. We’re craving dopamine.
Inhibiting a neural response is just as important as activating one when it comes to “executive” brain function. Examples of things that bind to inhibitory synapses are sedatives such as barbiturates, alcohol, and antihistamines. Synapses will be critical in our discussion of the adolescent brain because both the number and the type of synapses in our brains change as we age. They also change in relation to the amount of stimulation our brains experience. One topic that will come up later is the effect of illegal and illicit drugs and alcohol on these synapses, which we will cover in the chapter on addiction.
A popular instrument used by researchers to test inhibition is the Go/No-Go task in which subjects are told to press a button (the “Go” response) when a certain letter or picture appears, and not to press it (the “No-Go” response) when the letter X appears. Several studies have shown that children and adolescents generally have the same accuracy, but the reaction times, the speed at which a subject successfully inhibits a response, dramatically decrease with age in subjects age eight to twenty. In other words, it takes longer for adolescents to figure out when not to do something.
Signals move from one area of the brain to another along fiber tracks, and some of these tracks travel down through the core regions of the brain in order to send signals to and from the spinal cord. Brains are intricately interconnected by these fibers, and research using special brain scans is rapidly evolving to look at these connections. Because axons are designed to have a rapid pulse of electricity run through them to the connection point at the synapse, they act like electrical wires conducting an electrical signal. And just as an electrical wire needs insulation in order for the electricity not to dissipate along its length, so do the axons. Since we don’t have rubber in our brains, our axons are coated with a fatty substance called myelin. (See Figure 6.) The brain requires myelin in order to function normally, to get a signal from one region of the brain to another and also down to the spinal cord. As we said before, myelin is made by oligodendrocytes, and has a white hue due to its fatty content: hence the term “white matter.” By essentially “greasing” the “wires,” myelin allows signals to travel down axons faster, increasing the speed of a neural transmission as much as a hundredfold. Myelin also aids the speed of transmission by helping to cut down the synapses’ recovery time between neural firings, thereby allowing a thirtyfold increase in the frequency with which neurons transmit information. The combination of increased speed and decreased recovery time has been estimated by researchers as roughly equivalent to a three-thousand-fold increase in computer bandwidth. (Myelin also is the target of attack in the disease multiple sclerosis, or MS. Patients with MS have areas of inflammation in their white matter that come and go, and this is why they can lose functions like walking, sometimes only temporarily until the inflammation passes.)
At birth, a baby’s cortex contains little myelin; this explains why the electrical transmissions are so sluggish and an infant’s reaction times so slow. However, the baby’s brainstem is almost as fully myelinated as an adult’s, so it can control automatic functions like breathing, heartbeat, and gastrointestinal function necessary to stay alive. Connections to and from many other areas of the brain occur after birth, beginning with the motor and sensory areas at the bottom and back of the brain. As these areas become wired with myelin, infants are better able to process basic information from their senses—their eyes, ears, mouth, skin, and nose. Within the first year, the neural tracts that support brain regions involved in vision and other primary senses, as well as those involved in gross motor activity, are completed. This is, in part, why it takes about a year for a baby to become coordinated enough to walk. Much of the brain becomes insulated by age two, and high-level areas involved in language and fine motor coordination follow over the next few years when children are particularly primed to learn to talk and improve their fine motor skills. The more complex areas of the brain, especially the frontal lobes, take much, much longer and are not finished until a person is well into his or her twenties.
All of this learning is dependent on excitation, the driving force in our brains. Excitatory signals between neurons build brain connections and are required for brain development. Excitation can come from outside or inside your brain, but regardless, if a particular pathway of cells and their synapses are activated repeatedly, the synapses between them strengthen. Thus, cells that “fire” together “wire” together.
In the developing brain, especially in early childhood, as groups and pathways of neurons and their synapses get activated, the process of excitation “turns on” the molecular machinery in the cell. This actually results in the building of more synapses, a process we term synaptogenesis (birth of synapses). Synapses are increased in infancy through adolescence, peaking in early childhood. Because synaptogenesis is so dependent upon brain cells being activated by one another, a child’s brain has more excitatory than inhibitory neurotransmitters and synapses compared with an adult’s brain, where there is more balance between the two.
Excitation is a key element of learning. The period in early life in which excitation is so prominent is also called the “critical period,” when learning and memory are more robust than in later life. This allows the brain to be very sensitive to excitation and grow. Unfortunately, the abundant excitation in the developing brain carries a price: the risk for overexcitation. This explains why diseases that are a result of overexcitation, like epilepsy, are more common in childhood than adulthood. Seizures are the main symptom in epilepsy, and they are caused by too many brain cells turning on together without enough inhibition to balance them.
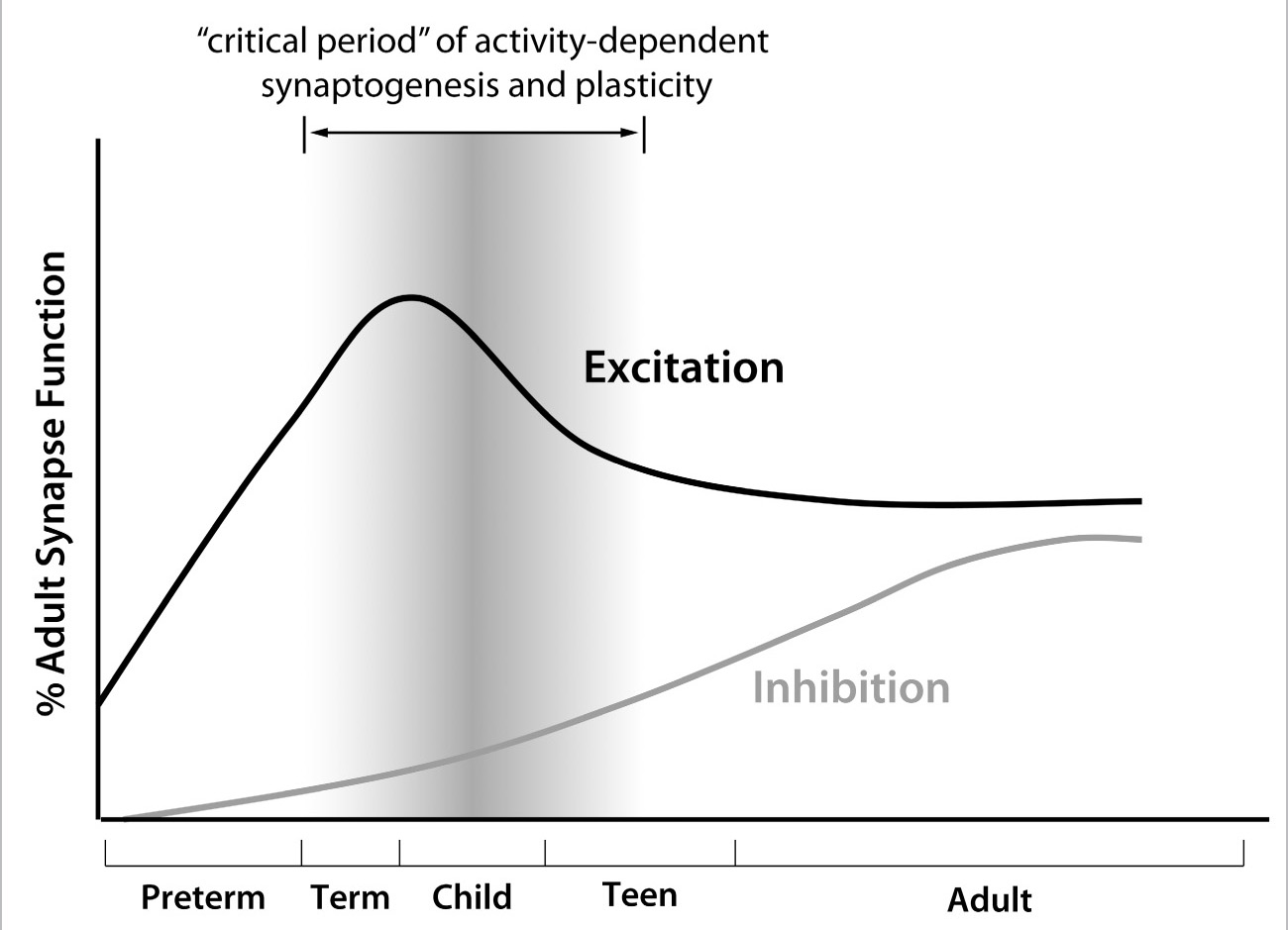
FIGURE 8. The Young Brain Has More Excitatory Synapses Than Inhibitory Synapses: The number of synapses increases from infancy through adolescence, peaking in early childhood.
Arborization, or the branching out of neurons, peaks in the first few years of life but continues, as we’ve seen, into adolescence. Gray matter density peaks in girls at age eleven and in boys at age fourteen, and waxes and wanes throughout adolescence.
White matter, or myelin, however, has only one trajectory in adolescence: up. Jay Giedd and colleagues at the National Institute of Mental Health scanned the brains of nearly one thousand healthy children, ages three to eighteen, and discovered this pattern of wiring. As we saw in Figure 4, researchers at the University of California, Los Angeles, built on those findings and compared the scans of young adults, ages twenty-three to thirty, with those of teenagers, ages twelve to sixteen. They found that myelin continues to be produced well past adolescence and even into a person’s thirties, making the communication between brain areas ever more efficient.
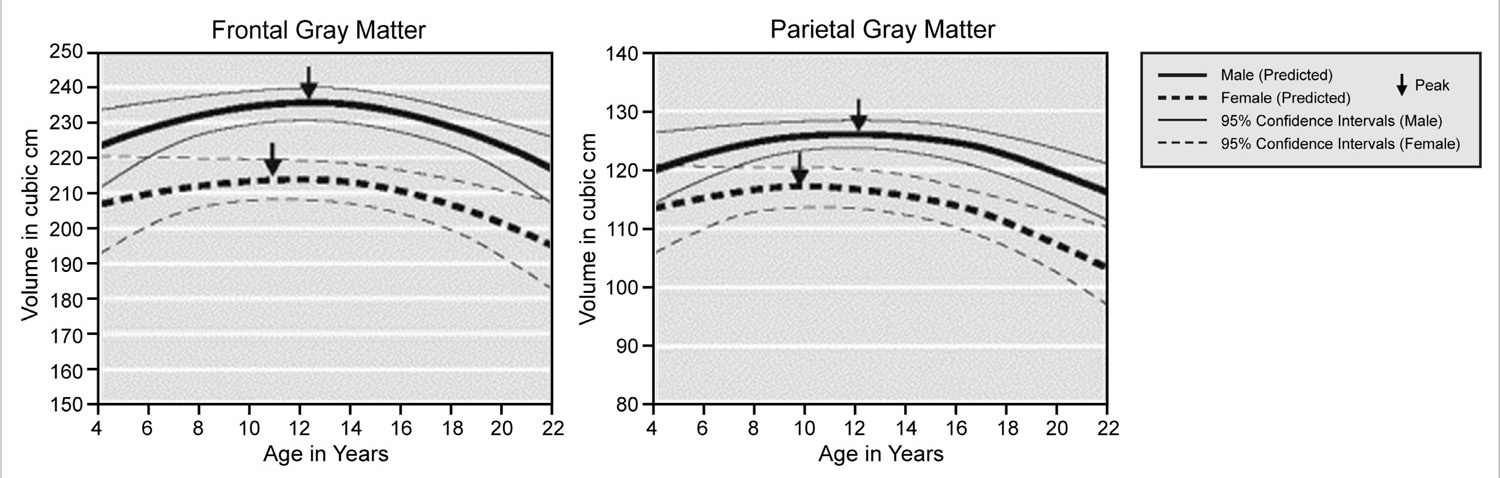
FIGURE 9. Gender Differences in Rate of Cortical Gray Matter Growth: Like the body, the male brain is on average larger than the female brain. Rates of growth in male and female brains also are different. In females, the growth rate of two areas important for cognitive maturity—the frontal lobes and the parietal lobes—peaks in the early teen years, but in males the peak does not occur until the late teens.
Without those insulated connections, a signal from one area of the brain, say fear and stress coming from the amygdala, has trouble linking up with another part of the brain, for instance the frontal cortex’s sense of judgment. For adolescents whose brains are still being wired, this means they sometimes find themselves in dangerous situations, not knowing what they should do next. This was confirmed scientifically in a 2010 study conducted by the British Red Cross into how teenagers react to emergencies involving a friend drinking too much alcohol. More than 10 percent of all children and young teens between the ages of eleven and sixteen have had to cope at one time or another with a friend who was sick, injured, or unconscious owing to excessive alcohol consumption. Half of those had to deal with a friend who passed out. More broadly, the survey found that nine out of ten adolescents have had to deal with some kind of crisis involving another person during their teenage years—a head injury, choking, an asthma attack, an epileptic seizure, etc. Forty-four percent of the teens surveyed admitted to panicking in that emergency situation, and nearly half (46 percent) acknowledged they didn’t know how to respond to the crisis at all.
Dan Gordon, a fifteen-year-old boy from Hampshire, England, who was interviewed by the Guardian for a story about the study, spoke about a house party he attended at which there was widespread underage drinking. After one girl passed out on the floor, facedown, she began to vomit, and the others in the room, all teenagers, panicked. Thinking only that they needed to prevent her from choking, they stood her up and, with effort, walked her outside for fresh air and waited for her to wake up. Dan admitted to the reporter that neither he nor anyone else at the party had thought to call for an ambulance. In other words, the teenagers’ amygdalae had signaled danger, but their frontal lobes didn’t respond. Instead, the teens acted in the moment.
My son Andrew witnessed something similar during college. He was visiting his then-girlfriend at a college in Boston. The girlfriend’s roommate also had an out-of-town visitor, a shy freshman girl from the South who quickly became intoxicated at a party in another student’s room. When Andrew and his girlfriend returned to her dorm, they found the young girl passed out, and just as in Dan Gordon’s story, they all panicked. Instead of calling 911 or campus security, or driving her to an emergency room, they found a couple of friends to help, and then drove all the way out to our house, about ten miles away.
“We didn’t want to call campus security,” Andrew’s girlfriend explained, as I observed the young girl, whom they had helped into the house and who was now almost unresponsive. “She’s a freshman. If we brought her to the health center, me and my roommate could get in trouble.”
Andrew and his former girlfriend were both twenty-one at the time, but the visiting student was just eighteen.
“What about taking her to the hospital?” I said.
“We didn’t know how drunk she was,” the other friend said. “She was talking when we put her in the car, and now she’s completely out of it.”
None of them in fact knew the girl—they had met her briefly for the first time earlier that day, when she had arrived to visit the roommate. She had her wallet and an ID from her South Carolina college with her, but no other information. The roommate who had invited her to Boston was nowhere to be found. Already drowsy, she was rapidly becoming more sedated, and then she vomited on the floor. At that point, I insisted they get her to a local community hospital just a mile from our house. It took three of them to half-carry her back to the car. About fifteen minutes later, I got a call from Andrew’s girlfriend, who said the hospital was going to admit the girl for observation. The poor thing spent an unhappy night in the hospital, and the college crew picked her up the next afternoon. On their way back to Boston, they stopped by my house to gather things they had left there the night before. The young freshman looked pale and very tired, but otherwise was fine. Apparently her blood alcohol level had peaked at 0.34, which was more than four times the legal driving limit, and life threatening. Had she not been taken to the hospital, where her stomach was pumped and charcoal administered to prevent her body from absorbing any more alcohol, I shudder to think of what might have happened. As I had a captive audience, I sat them all down in the kitchen, turned on my laptop, and showed them a chart about blood alcohol levels and the effects on coordination and consciousness. I pointed out that 0.4, which was only a little more than her blood alcohol reached at its height, can be lethal. Turns out she had done about seventeen Jell-O shots that evening—to the best of her memory. There was no point in asking the usual question—“What were you thinking?”—but I felt this was a good teaching moment to show them all how close she had come to a very different end the night before.
The young girl recovered and hopefully learned her lesson, but obviously the consequences of poor decision-making can be, and often are, disastrous for teens. Bennett Barber was sixteen years old on New Year’s Eve 2008 when he left an unsupervised party at a friend’s house in Marblehead, Massachusetts, and began to walk home. It was around 11:30 p.m., snow was falling, and the wind was gusting up to thirty miles per hour. Dressed in jeans and sneakers, Bennett was drunk and disoriented, and although his home was only a half mile away, he became lost. With the temperature plunging into single digits, Bennett eventually collapsed, face-first, into a snowbank. At three o’clock in the morning his mother notified the police, and a search party was sent out into the subfreezing night. Hours later, a firefighter discovered a beer bottle in the snow and followed a blurry set of footprints. When he found Bennett, the boy was semiconscious and suffering from hypothermia. He was also missing a sneaker and a sock. The high school hockey player was taken by ambulance to Massachusetts General Hospital, where his core temperature was only 88 degrees and his right foot appeared to be frozen solid. Isolated in a special chamber to raise his body temperature, he was eventually transferred to a burn center for treatment of his frostbite.
Bennett later told his father why it had taken so long for authorities to rescue him. He was trying to elude them, he said. The police report filled in the details:
He remembers seeing all the lights, but told his father that he hid every time someone with a light went by, because he did not want to get in trouble for drinking.
The teenage girl who hosted the spontaneous party when her parents went out for the night initially told the police that Bennett was drunk when he arrived and that she had walked him part of the way home. Not until 5:00 a.m. did she admit the truth, that there had been more than a dozen people at the house, many of them drinking alcohol, all underage, and that she tried to clear them out around 11:30 before her parents returned home. Two girls said they were going to walk Bennett up the street, “but when they went outside with him and he was too drunk,” they took him back inside and left him alone while they helped their friend clean up. That was the last time they saw Bennett.
Teen consumption of alcohol was only half the problem. The other half was the poor decision-making on the part of Bennett and his friends at the party, the lying that led to a delay before the police found Bennett, and even his panic at the thought of being caught by the police. All the teenagers involved exhibited a stunning lack of insight.
What scientists tell us is that insight depends on the ability to look outside oneself, and because that skill arises in the frontal and prefrontal lobes, it takes time to develop. The dynamic changes taking place in the brain are part of what make the adolescent years an age of exuberance. But a malleable, still-maturing teenage brain can be a scary proposition. Anything can happen—much of it not good. Teenagers may look like adults, they may even think like adults in many ways, and their ability to learn is staggering, but knowing what teenagers are unable to do—what their cognitive, emotional, and behavioral limitations are—is critically important.
4
Learning A Job for the Teen Brain
What did I do wrong?”
Often that’s the second question I get from parents of teenagers. The first question is usually rhetorical: