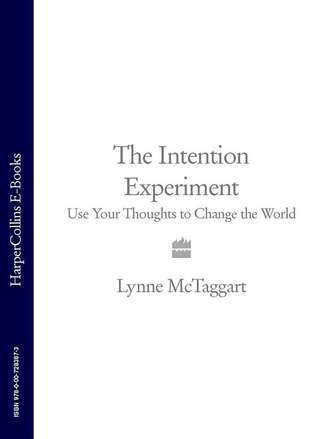
Полная версия
The Intention Experiment: Use Your Thoughts to Change the World
21. J. Hasted, The Metal Benders, London: Routledge & Kegan Paul, 1981, as cited in W. Tiller, Science and Human Transformation; Subtle Energies, Intentionality and Consciousness, Walnut Creek, Calif.: Pavior Publications, 1997: 13.
22. McTaggart, The Field, op. cit.: 199.
23. W. W. Monafo and M. A. West, ‘Current recommendations for topical burn therapy’, Drugs, 1990; 40: 364–73.
PART ONE
The Science of Intention
A human being is part of the whole, called by us
‘universe’, a part limited in time and space. He
experiences himself, his thoughts and feelings as
something separated from the rest – a kind of
optical delusion of his consciousness.
Albert Einstein
CHAPTER ONE
Mutable Matter
FEW PLACES IN THE GALAXY are as cold as the helium-dilution refrigerator in Tom Rosenbaum’s lab. Temperatures in the refrigerator – a boiler-sized circular apparatus with a number of cylinders – can descend to a few thousandths of a degree above absolute zero, almost 273°C below freezing – three thousand times colder than the farthest reaches of outer space. For two days, liquid nitrogen and helium circulate around the refrigerator, and then three pumps constantly blasting out gaseous helium take the temperature down to the final rung. Without heat of any description, the atoms in matter slow to a crawl. At this scale of coldness, the universe would grind to a halt. It is the scientific equivalent of hell freezing over.
Absolute zero is the preferred temperature of a physicist like Tom Rosenbaum. At 47, as a distinguished professor of physics at the University of Chicago and former head of the James Franck Institute, Rosenbaum was in the vanguard of experimental physicists who liked exploring the limits of disorder in condensed-matter physics, the study of the inner workings of liquids and solids when their underlying order was disturbed.1 In physics, if you want to find out how something behaves, the best way is simply to make it uncomfortable and then see what happens. Creating disorder usually involves adding heat or applying a magnetic field to determine how it will react when disturbed and also to determine which spin position – or magnetic orientation – the atoms will choose.
Most of his colleagues in condensed-matter physics remained interested in symmetrical systems such as crystalline solids, whose atoms are arranged in orderly array, like eggs in a carton, but Rosenbaum was drawn to strange systems that were inherently disordered – to which more conventional quantum physicists referred disparagingly as ‘dirt’. In dirt, he believed, lay exposed the unprobed secrets of the quantum universe, uncharted territory that he was happy to navigate. He loved the challenge posed by spin glasses, strange hybrids of crystals, with magnetic properties, technically considered slow-moving liquids. Unlike a crystal, whose atoms point in the same direction in perfect alignment, the tiny magnets associated with the atoms of a spin glass are wayward and frozen in disarray.
The use of extreme coldness allowed Rosenbaum to slow down the atoms of these strange compounds enough to observe them minutely, and to tease out their quantum mechanical essence. At temperatures near to absolute zero, when their atoms are nearly stationary, they begin taking on new collective properties. Rosenbaum was fascinated by the recent discovery that systems disorderly at room temperature display a conformist streak once they are cooled down. For once, these delinquent atoms begin to act in concert.
Examining how molecules behave as a group in various circumstances is highly instructive about the essential nature of matter. In my own journey of discovery, Rosenbaum’s laboratory seemed the most appropriate place to begin. There, at those lowest temperatures where everything occurs in slow motion, the true nature of the most basic constituents of the universe might be revealed. I was looking for evidence of ways in which the components of our physical universe, which we think of as fully realized, are capable of being fundamentally altered. I also wondered whether it could be shown that quantum behaviour like the observer effect occurs outside the subatomic world, in the world of the everyday. What Rosenbaum had discovered in his refrigerator might offer some vital clues as to how every object or organism in the physical world, which classical physics depicts as an irreversible fact, a finalized assemblage only changeable by the brute force of Newtonian physics, could be affected and ultimately altered by the energy of a thought.
According to the second law of thermodynamics, all physical processes in the universe can only flow from a state of greater to lesser energy. We throw a stone into a river and the ripple it makes eventually stops. A cup of hot coffee left standing can only grow cold. Things inevitably fall apart; everything travels in a single direction, from order to disorder.
But this might not always be inevitable, Rosenbaum believed. Recent discoveries about disordered systems suggested that certain materials, under certain circumstances, might counteract the laws of entropy and come together rather than fall apart. Was it possible that matter could go in the opposite direction, from disorder to greater order?
For ten years Rosenbaum and his students at the James Franck Institute had been asking that question of a small chunk of lithium holmium fluoride salt. Inside Rosenbaum’s refrigerator lay a perfect chip of rose-coloured crystal, no bigger than the head of a pencil, wrapped in two sets of copper coils. Over the years, after many experiments with spin glasses, Rosenbaum had grown very fond of these dazzling little specimens, one of the most naturally magnetic substances on earth. This characteristic presented the perfect situation in which to study disorder, but only after he had altered the crystal beyond recognition into a disordered substance.
He had first instructed the laboratory that grew the crystals to combine the holmium with fluorine and lithium, the first metal on the periodic table. The resulting lithium holmium fluoride salt was compliant and predictable – a highly ordered substance whose atoms behaved like a sea of microscopic compasses all pointing north. Rosenbaum then had wreaked havoc on the original salt compound, instructing the lab to rip out a number of the atoms of holmium, bit by bit, and replace them with yttrium, a silvery metal without such natural magnetic attraction, until he was left with a strange hybrid of a compound: a salt called lithium holmium yttrium tetrafluoride.
By virtually eliminating the magnetic properties of the compound, Rosenbaum eventually had created spin-glass anarchy – the atoms of this Frankenstein monstrosity pointing any way they liked. Being able to manipulate the essential property of elements like holmium by creating weird new compounds so cavalierly was a little like having ultimate control over matter itself. With these new spin-glass compounds, Rosenbaum could virtually change the properties of the compound at will; he could make the atoms orientate in a particular direction, or freeze them in some random pattern.
Nevertheless, his omnipotence had a limit. Rosenbaum’s holmium compounds behaved themselves in some regards, but not in others. One thing he could not do was to get them to obey the laws of temperature. No matter how cold Rosenbaum made his refrigerator, the atoms inside them resisted any sort of ordered orientation, like an army refusing to march in step. If Rosenbaum was playing God with his spin glasses, the crystal was Adam, stubbornly refusing to obey His most fundamental law.
Sharing Rosenbaum’s curiosity about the strange property of the crystal compound was a young student called Sayantani Ghosh, one of his star PhD candidates. Sai, as her friends called her, a native of India, had graduated with a first-class honours degree from Cambridge, after which she had chosen Tom’s lab for her doctoral programme in 1999. Almost immediately, she had distinguished herself by winning the Gregor Wentzel Prize, given each year by the University of Chicago’s physics department to the best first-year graduate student teaching assistant. The slight 23-year-old, who at first glance appeared abashed, hiding behind her copious dark hair, had soon impressed her peers and teachers alike with her bold authority, a rarity among science students, and her ability to translate complex ideas to the level an undergraduate could comprehend. Sai shared the distinction of winning the coveted prize with only one other woman since its inception 25 years before.
According to the laws of classical physics, applying a magnetic field will disrupt the magnetic alignment of a substance’s atoms. The degree to which this happens is the salt’s ‘magnetic susceptibility’. The usual pattern with a disordered substance is that it will respond to the magnetic field for a time and then plateau and tail off, as the temperature drops or the magnetic field reaches a point of magnetic saturation. The atoms will no longer be able to flip in the same direction as that of the magnetic field and so will begin to slow down.
In Sai’s first experiments, the atoms in the lithium holmium yttrium salt, as predicted, grew wildly excited with the application of the magnetic field. But then, as Sai increased the field, something strange began to happen. The more she turned up the frequency, the faster the atoms continued to flip over. What is more, all the atoms, which had been in a state of disarray, began pointing in the same direction and operating as a collective whole. Then, small clusters of about 260 atoms aligned, forming ‘oscillators’, spinning collectively in one direction or another. No matter how strong the magnetic field that Sai applied, the atoms remained stubbornly aligned with each other, acting in concert. This self-organization persisted for 10 seconds.
At first, Sai and Rosenbaum thought these effects might have something to do with the strange effects of the remaining atoms of holmium, known to be one of the very few substances in the world with such long-range internal forces that in some quarters it was described and worked out mathematically as something existing in another dimension.2 Although they didn’t understand the phenomenon they had observed, they wrote up their results, which were published in the journal Science in 2002.3
Rosenbaum decided to carry out another experiment to attempt to isolate the property in the crystal’s essential nature that had enabled it to override such strong outside influences. He left the study’s design to his bright young graduate student, suggesting only that she create a computerized three-dimensional mathematical simulation of the experiment she had intended to carry out. In experiments of this nature on such tiny matter, physicists must rely on a computerized simulation to confirm mathematically the reactions they are witnessing experimentally.
Sai spent months developing the computer code and building her simulation. The plan was to find out a bit more about the salt’s magnetic capability, by applying two systems of disorder to the crystal chip: higher temperatures and a stronger magnetic field.
She prepared the sample by placing it in a little 2.4 x 4.8 cm copper holder, then wrapped two coils around the tiny crystal: one a gradiometer, to measure its magnetic susceptibility and the direction of spin of the individual atoms, and the other to cancel out any random flux affecting the atoms inside.
A connection attached to her PC would enable her to change the voltage, the magnetic field or the temperature, and would record any changes whenever she altered one of the variables by the tiniest degree.
She began lowering the temperature, a fraction of a kelvin (K) at a time, and then began applying a stronger magnetic field. To her amazement, the atoms kept aligning progressively. Then she tried applying heat, and discovered they again aligned. No matter what she did, in every instance the atoms ignored the outside interference. Although she and Tom had flushed out most of the compound’s magnetic component, of its own volition, as it were, it was turning into a larger and larger magnet.
That’s weird, she thought. Perhaps she should take more data, just to ensure they had encountered nothing strange in the system.
She repeated her experiment over six months until the early spring of 2002, when her computer simulation was finally complete. One evening, she mapped the results of the simulation on a graph, and then she superimposed the results from her actual experiment. It was as though she had drawn a single line. There on the computer screen was a perfect duplicate: the diagonal line formed from the computer simulation lay exactly over the diagonal line created from the results of the experiment itself. What she had witnessed in the little crystal was not an artefact, but something real that she had now reproduced in her computer simulation. She had even mapped out where the atoms should have been on the graph, had they been obeying the usual laws of physics. But there they were in a line: a law completely unto themselves.
She wrote Rosenbaum a guarded email late that evening: ‘I’ve got something interesting to show you in the morning.’ The following day, they examined her graph. There was no other possibility, they both realized; the atoms had been ignoring her and instead were controlled by the activity of their neighbours. No matter whether she blasted the crystal with a strong magnetic field or an increase in temperature, the atoms overrode this outside disturbance.
The only explanation was that the atoms in the sample crystal were internally organizing and behaving like one single giant atom. All the atoms, they realized with some alarm, must be entangled.
One of the strangest aspects of quantum physics is a feature called ‘non-locality’, also poetically referred to as ‘quantum entanglement’. The Danish physicist Niels Bohr discovered that once subatomic particles such as electrons or photons are in contact, they remain cognizant of and influenced by each other instantaneously over any distance forever, despite the absence of the usual things that physicists understand are responsible for influence, such as an exchange of force or energy. When entangled, the actions – for instance, the magnetic orientation – of one will always influence the other in the same or the opposite direction, no matter how far they are separated. Erwin Schrödinger, another one of the original architects of quantum theory, believed that the discovery of non-locality represented no less than quantum theory’s defining moment – its central property and premise.
The activity of entangled particles is analogous to a set of twins being separated at birth, but retaining identical interests and a telepathic connection forever. One lives in Colorado, and the other in London. Although they never meet again, both like the colour blue. Both take a job in engineering. Both like to ski; in fact when one falls down and breaks his right leg at Vale, his twin breaks his right leg at precisely that moment, even though he is 4000 miles away, sipping a latte at Starbucks.4 Albert Einstein refused to accept non-locality, referring to it disparagingly as ‘spukhafte Fernwirkungen’ or ‘spooky action at a distance’. This type of instantaneous connection would require information travelling faster than the speed of light, he argued through a famous thought experiment, which would violate his own special relativity theory.5 Since the formulation of Einstein’s theory, the speed of light (299,792,458 metres per second) has been used as the absolute limiting factor on how quickly one thing can affect something else. Things are not supposed to be able to affect other things faster than the time it would take the first thing to travel to the second thing at the speed of light.
Nevertheless, modern physicists, such as Alain Aspect and his colleagues in Paris, have demonstrated decisively that the speed of light is not an absolute outer boundary in the subatomic world. Aspect’s experiment, which concerned two photons fired off from a single atom, showed that the measurement of one photon instantaneously affected the position of the second photon6 so that it has the same or opposite spin or position (as IBM physicist Charles H. Bennett once put it, ‘opposite luck’).7 The two photons continued to talk to each other and whatever happened to one was identical to, or the very opposite of, what happened to the other. Today, even the most conservative physicists accept non-locality as a strange feature of subatomic reality.8
Most quantum experiments incorporate some test of Bell’s Inequality. This famous experiment in quantum physics was carried out by John Bell, an Irish physicist who developed a practical means to test how quantum particles really behaved.9 This simple test required that you get two quantum particles that had once been in contact, separate them and then take measurements of the two. It is analogous to a couple named Daphne and Ted who have once been together but are now separated. Daphne can choose one of two possible directions to go in and so can Ted. According to our commonsense view of reality, Daphne’s choice should be utterly independent of Ted’s.
When Bell carried out his experiment, the expectation was that one of the measurements would be larger than the other – a demonstration of ‘inequality’. However, a comparison of the measurements showed that both were the same and so his inequality was ‘violated’. Some invisible wire appeared to be connecting these quantum particles across space, to make them follow each other. Ever since, physicists have understood that when a violation of Bell’s Inequality occurs, it means that two things are entangled.
Bell’s Inequality has enormous implications for our understanding of the universe. By accepting non-locality as a natural facet of nature we are acknowledging that two of the bedrocks on which our world view rests are wrong: that influence only occurs over time and distance, and that particles like Daphne and Ted, and indeed the things that are made up of particles, only exist independently of each other.
Although modern physicists now accept non-locality as a given feature of the quantum world, they console themselves by maintaining that this strange, counter-intuitive property of the subatomic universe does not apply to anything bigger than a photon or electron. Once things got to the level of atoms and molecules, which in the world of physics is considered ‘macroscopic’, or large, the universe started behaving itself again, according to predictable, measurable, Newtonian laws.
With one tiny thumbnail’s worth of crystal, Rosenbaum and his graduate student demolished that delineation. They had demonstrated that big things like atoms were non-locally connected, even in matter so large you could hold it in your hand. Never before had quantum non-locality been demonstrated on such a scale. Although the specimen had been only a tiny chip of salt, to the subatomic particle, it was a palatial country mansion, housing a billion billion (1,000,000,000,000,000,000 or 1018) atoms. Rosenbaum, ordinarily loathe to speculate about what he could not yet explain, realized that they had uncovered something extraordinary about the nature of the universe. And I realized they had discovered a mechanism for intention: they had demonstrated that atoms, the essential constituents of matter, could be affected by non-local influence. Large things like crystals were not playing by the grand rules of the game, but by the anarchic rules of the quantum world, maintaining invisible connections without obvious cause.
In 2002, after Sai wrote up their findings, Rosenbaum polished up the wording and sent off their paper to Nature, a journal notorious for conservatism and exacting peer review. After four months of responding to the suggestions of reviewers, Ghosh finally got her paper published in the world’s premier scientific journal, a laudatory feat for a 26-year-old graduate student.10
One of the reviewers, Vlatko Vedral, noted the experiment with a mix of interest and frustration.11 A Yugoslav who had studied at Imperial College, London, during his country’s civil war and subsequent collapse, Vedral had distinguished himself in his adopted country and been chosen to head up quantum information science at the University of Leeds. Vedral, who was tall and leonine, was part of a small group in Vienna working on frontier quantum physics, including entanglement.
Vedral first theoretically predicted the effect that Ghosh and Rosenbaum eventually found three years later. He had submitted the paper to Nature in 2001, but the journal, which preferred experiment to theory, had rejected it. Eventually, Vedral managed to publish his paper in Physical Review Letters, the premier physics journal.12 After Nature decided to publish Ghosh’s study, its editors threw him a conciliatory bone. They allowed him to be a reviewer on the paper, and then offered him a place in the same issue to write an opinion piece on the findings.
In the article, Vedral allowed himself some speculation. Quantum physics is accepted as the most accurate means of describing how atoms combine to form molecules, he wrote, and since molecular relationship is the basis of all chemistry, and chemistry is the basis of biology, the magic of entanglement could well be the key to life itself.13
Vedral and a number of others in his circle did not believe that this effect was unique to holmium. The central problem in uncovering entanglement is the primitive state of our technology; isolating and observing this effect is only possible at the moment by slowing atoms down so much in such cold conditions that they are hardly moving. Nevertheless, a number of physicists had observed entanglement in matter at 200 K, or –73°C – a temperature that can be found on Earth in some of its very coldest places.
Other researchers have proved mathematically that everywhere, even inside of our own bodies, atoms and molecules are engaged in an instantaneous and ceaseless passing back and forth of information. Thomas Durt of Vrije University in Brussels demonstrated through elegant mathematical formulations that almost all quantum interactions produce entanglement, no matter what the internal or surrounding conditions. Even photons, the tiniest particles of light emanating from stars, are entangled with every atom they meet on their way to earth.14 Entanglement at normal temperatures appears to be a natural condition of the universe, even in our bodies. Every interaction between every electron inside of us creates entanglement. According to Benni Reznik, a theoretical physicist at Tel Aviv University in Israel, even the empty space around us is heaving with entangled particles.15
The English mathematician Paul Dirac, an architect of quantum field theory, first postulated that there is no such thing as nothingness, or empty space. Even if you tipped all matter and energy out of the universe and examined all the ‘empty’ space between the stars you would discover a netherworld world teeming with subatomic activity.
In the world of classical physics, a field is a region of influence, in which two or more points are connected by a force, like gravity or electromagnetism. However, in the world of the quantum particle, fields are created by exchanges of energy. According to Heisenberg’s uncertainty principle, one reason that quantum particles are ultimately unknowable is because their energy is always being redistributed in a dynamic pattern. Although often rendered as tiny billiard balls, subatomic particles more closely resemble little packets of vibrating waves, passing energy back and forth as if in an endless game of basketball. All elementary particles interact with each other by exchanging energy through what are considered temporary or ‘virtual’ quantum particles. These are believed to appear out of nowhere, combining and annihilating each other in less than an instant, causing random fluctuations of energy without any apparent cause. Virtual particles, or negative energy states, do not take physical form, so we cannot actually observe them. Even ‘real’ particles are nothing more than a little knot of energy, which briefly emerge and disappear back into the underlying energy field.