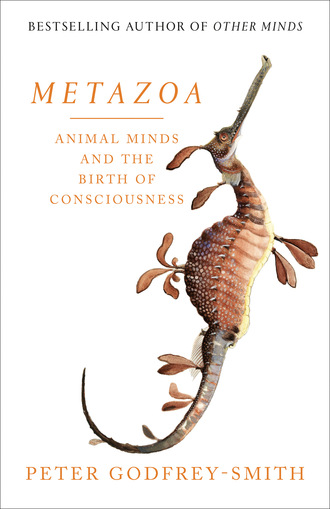
Полная версия
Metazoa
It is worth pausing to think about that combination of features—being simple and being far from us. There is no general reason why these should be associated. We might have found, on Earth today, an extremely complicated animal whose evolutionary path turned away from ours very early. All the time we have had to evolve our complex bodies and brains is time that this other animal would have had, too. The best partial example of this combination—complicated, far from us—is the octopus, waiting in a later chapter of this book. But octopuses are not nearly as far from us as sponges and the other animals we are talking about now.
It has often been tempting to tell a story in which some of our earliest animal ancestors looked like a sponge, then later ancestors looked like a jellyfish, and so on. That sequence is not impossible, but it can’t just be read off the evolutionary tree. To do this would be to treat a set of cousins as if they were grandparents—or to treat distant cousins as if they looked more like grandparents than other cousins do. Once we put the point in terms of cousins and grandparents, it is clear that this reasoning would not make sense. However, there can be other reasons that some particular distant cousins might contain clues.
We, in our bodies, possess various evolutionary inventions (brains, hearts, backbones, and so on) that had to come into existence somehow. Sponges and jellyfish live without those inventions, though they do share ancestors with us. So these animals show, first of all, what you might be like if you had to live without them. Further, these animals are not part of evolutionary lines that had these features at some stage but lost them; it is pretty clear they never had them. The inventions they lack include more than mere accessories, too. The left-right body layout that we have is an invention. The intricate folds of tissue that make up our internal organs are an invention. Looking at distant animals who lack these inventions, along with genetic evidence and fossils, we can start to get a sense of what ancestors far below us on the tree might have looked like.
Light Through Glass
Historically, sponges have been seen as the most important of the living clues to very early animals. Sponges have a decent fossil record and are the best known. So without making assumptions about whether the sponges around now look much like any of our ancestors, instead approaching them just as their unusual selves, let’s take a closer look.
Sponges extend widely through the seas, from fingers and trees in temperate waters and huge funnels on tropical reefs to the freezing deep-sea towers that head this chapter. Some encrust themselves on other organisms rather than growing a shape of their own. Their modus vivendi in many cases is that water is drawn into their lower parts, passed upward through the body, and exits at the top. Food, mainly bacteria, is taken from the water as it passes through. A few sponges have a more ambitious diet; the deep sea has some predatory sponges that trap and consume small animals.
A sponge has a body, but one with a big difference from bodies like ours. Most of its cells are in direct contact with the water moving through it. The sponge body is a maze of fine passageways, lined with microbial partners, and the environment suffuses that body.
A sponge has no brain or other nervous system. The larvae (immature forms), which look like tiny fat cigars, can swim, and they have some sensing structures that resemble parts of a nervous system. These sensing mechanisms are facing out to the world, not toward other cells in the same body. The larva settles and the adult grows up in place. Though a sponge has no nervous system, it is not inert. Inside each cell is the storm described earlier in this chapter. A sponge as a whole seems a good deal quieter, but it has something of an active side.
Water moving through the sponge’s body is actively pumped by cells with little tails (flagella). This pumping can be modified or stopped, especially if the water is dirty and the sponge’s channels risk becoming clogged. For a collection of cells with no nervous system, this is not easily achieved. There appear to be specialized sensory cells along the chimneys through which water passes, and these cells signal to others. Given what cells are, it is a significant task for one to influence another. The usual way this is done is with the release of small molecules from one cell, taken up by others. The result is to contract, squeeze down, the canals. This process is slow, but there is little need for it to be fast. In some cases, the sponge expands a little first and then contracts, in a drowsy and invisible “sneeze.”
All this is a reminder of both the opportunites and difficulties of multicellular life. A cell within a sponge is at little risk of being eaten by a larger cell, as it would be if it floated freely in the water. If the cell was merely locked in place with others, though, it could easily starve. In a sponge, a filigree of channels and chimneys keeps most cells in contact with water. But if something then has to be done, it is hard to achieve coordination, especially coordinated motion. All in all, the result looks a fair bit like a plant. Most sponges are entirely content with that, and have been doing what they do for many more years than us. Still, a few tried something different.
The Hexactinellida, or glass sponges, explore in their bodies this chapter’s themes of unity and selfhood in a unique way. A glass sponge is a multicellular organism, like other animals, but as it grows up, most of its cells fuse, losing their boundaries. They don’t abandon boundaries with the outside world, but with neighboring cells. The body eventually forms a single connected net, often described as a “three-dimensional cobweb,” draped over harder elements that support it.
Those harder elements are made of glass. Variously, according to species, they resemble daggers, stars, or snowflakes. These are grouped together to form flowers, vine clusters, and—finally, in combination—the skeleton that holds up the tower. (The drawings of these tiny parts below, by Rebecca Gelernter, reproduce engravings made from specimens collected on the nineteenth-century Challenger expedition, the voyage that doomed Bathybius.)
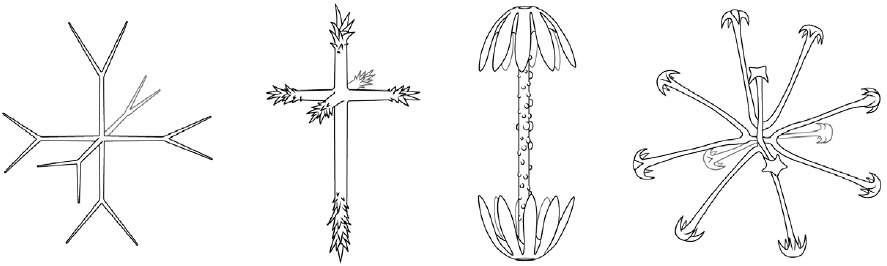
Like other sponges, the Hexactinellida live in rich associations with other kinds of life. The Venus’s flower basket is a glass sponge usually found with a pair of small shrimp living inside its body. These shrimp enter the sponge when they are very small, and grow up there, never leaving. Eventually they become too big to slip out through any opening. The shrimp eventually reproduce together. They keep the sponge clean, and benefit in turn from both the protection of the sponge’s skeleton and the food in the water the sponge draws through its body.
Glass sponges have no nervous system, but they are not electrically inert, and here the taming of charge takes an unusual form. With that fine living cobweb draped over a skeleton, they, uniquely among sponges, have electrical signaling and a kind of “action potential.” A glass sponge usually moves water through its body in a continual flow. In response to some stimuli, though, such as the plucking of a single glass element from its body, the pumping quickly ceases. This is done by sending an electrical pulse through the body. Electrically, a glass sponge behaves like a single enormous cell; a pulse can run uninterrupted over the whole. A glass sponge achieves coordination not by coordinating signals between cells, but by being, in large part, post-cellular. It is a product of the animal evolutionary path, but one that has partially abandoned the multicellular form of life, living by means of a different kind of unity.
I have been talking about charge, communication, and coordination in these creatures. But this is also an animal made largely of glass—not the charge-conducting cobweb, but the skeleton beneath. A notable feature of glass, of course, is how it handles light. Parts of the skeleton of some glass sponges resemble fiber-optic cable, along which light is conducted and filtered.
Is the sponge doing anything biologically significant with light, or is this conduction just a consequence of employing glass as a building material? Is light used, or just channeled around by happenstance? A wide and rather wonderful range of possibilities has been raised and discussed for different sponge species. Light, which except in the shallowest cases will derive mostly from bioluminescence of one kind or another, might be a further means for communication within the animal. It might also be feeding some of the microorganisms within: tiny diatoms and other creatures that live on light huddle deep inside some sponges, perhaps so deep that they could not get enough light to stay alive if the sponge did not channel rays to them. Light in these cases is even ducted a little way into the seafloor itself. The Venus’s flower basket emits light into the surrounding water, perhaps as a faint lamp in the ocean that attracts the shrimp who come to live inside. These possibilities are unresolved, and some biologists think the light in and around the sponges will be too weak to do much. Either by design or by accident, a glass sponge is a collector and curator of biological light.
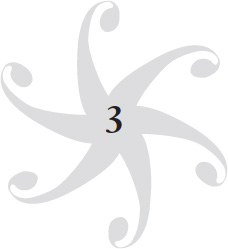
THE ASCENT OF SOFT CORAL
Ascent
In a bay north of Sydney, Australia, a short way from the stairs we walked down in Chapter 1, is a sandy underwater plain. The bay is formed where a river winding down from eucalyptus forests inland reaches the Pacific.
The underwater plain experiences a strong tidal flow. Water pushes hard up the river from the ocean as the tide rises, and runs back out to sea as it falls. This flow of water attracts a rich range of animals, but also means that the area can only be visited by scuba divers for a couple of hours each day, around the periods when the water comes to rest at the turn of the tide. There is about an hour at each pause. You dive on top of the high, until the water starts to run.
The turn of the tide comes on fast—a tug and then you are moving. Soon it is not possible to swim against the flow. If you stay too long, you can quickly be pulled out to sea.
A few areas on the plain have fields of purple and white soft coral. This coral is soft and wispy, not jagged and mineralized like tropical “hard” corals. The corals form trees that have cauliflower shapes, though a comparison to cauliflower does them no justice at all. From a distance they look like puffs of white and purple cloud; up close one sees delicate veins and filaments, with cowrie shells and crabs living among the branches.
If you approach on a slight current, perhaps riding the end of the incoming tide, it is like coming in on a glider, silently approaching clouds, and finding that the clouds are growing from the earth on stout pale stems. These trees are not single organisms, but colonies of many small animals, coral polyps. From Chapter 2 we know there is an unending frenzy of microscopic activity within them. But the corals seem to sit motionless while more active animals crawl among their branches.
A few years ago, a local diver and researcher, Tom Davis, who had dived in the bay countless times on the still of the high tide, wondered: What do the soft corals do when no one is looking? Through most of any day the tidal flow is too strong for a diver to be down there with them, but he could put cameras down, and take time-lapse footage of what goes on when the water is fast and no humans are around to see.
Diving with his wife, Nicola, he dropped cameras in various sites where the coral is found. When the cameras were retrieved and they watched the results, they found that as the waters sped up with each change of tide, the soft corals slowly rose, by inflating their bodies, until they were up to three times their still-water size. Very likely, they were stretching out to take in the extra food swept past on the tidal flow. The corals subsided when the waters slowed, and sat lower during the hour or so when humans might return.
In Search of the First Animal Actions
Corals are cnidarians (again with a silent “c,” so “nye-dairians”), the same group of animals that includes jellyfish and anemones. This group diverged from our own evolutionary line at a time still early in the history of animal life. A coral might have last shared an ancestor with you 650 or 700 million years ago. The date is unclear, but it was certainly more recent than the time you last shared an ancestor with a sponge.
A cnidarian body is soft, radial in organization—organized around a disc or cup—and often fringed with tentacles. These can be long streamers or short fingers. Inside the body is muscle, and the electrified threads of a nervous system.
Many cnidarians have a complicated life cycle, proceeding through a series of different bodily forms. These transitions are a bit like metamorphosis, as in caterpillar to butterfly, but not quite the same, as the bodies not only transform but multiply at more than one step; it is as if a caterpillar could make many butterflies, as well as a butterfly many caterpillars. The two adult forms a cnidarian can have are the polyp and the medusa. A polyp is usually fixed to a surface, often shaped like a cup. The medusa is the familiar jellyfish body, swimming in open water with streaming tentacles. Many cnidarians cycle between these two bodies. Corals and anemones only live as polyps.
A little way from the cloud-like trees on the plain, another kind of soft coral lives on a tangled reef. These corals sometimes form shrubs but also cluster in irregular masses. Each polyp looks like a white flower with eight long, finger-like tentacles. Each finger, in turn, has smaller rays extending from it sideways. Those are called pinnules. Fingers on fingers. A coral colony is often partly covered by an orange sponge, growing as a blanket and allowing the flower-like parts of the polyps to reach through.
Having eight of the finger-like tentacles, these animals are called “octocorals.” A mass of them together forms a forest of tiny hands. As you watch, if you are patient, often you will see a slow opening of a polyp like a reach, or a closing of the fingers, a clench.
Occasionally a single tentacle will curl and close while others stay extended. At other times the whole hand will close. On a larger scale, you might come across zones or outcrops where every hand is closed, while a neighboring zone has most hands open. They look like they are reaching and grasping for things, but for a while it was unclear what, if anything, they were catching. John Lewis, a Canadian biologist, looked at thirty octocoral species and found that some were indeed catching not only plankton, but tiny invertebrate animals. This talk of reaching and seizing suggests a momentary action that a person might make, but in general, the process occurs in a graceful slow motion: faster than a plant, but slower than the scale of familiar, busy animal behaviors. In these unfurlings and clenches, there are hints, indicators, echoes of the beginnings and simplest forms of animal action.
Why might this be? First, cnidarians are old animal forms, with body designs that are very possibly part of our past. It is not clear that any modern-day cnidarian—anemone, coral, jellyfish—closely resembles an ancestor of ours, but that radial layout probably does resemble the layout of some of our predecessors.
Second, they can act. Action itself was not invented by cnidarians. Many single-celled organisms can swim, with propeller-like flagella or hair-like cilia. Some can envelop prey and transform their shape. Stirrings into motion can be seen across all the candidates for early animal forms. In the previous chapter we saw the controlled pumping of water through sponges. This is akin to an action and it might be very old.
Evolution is full of gray areas and partial cases—the first of something is rarely clear. Evolution also sometimes sees the rediscovery of something old at a new level or scale. In single-celled life, action exists; there is swimming, seizing, and engulfing. These actions may have been an important spur to the evolution of multicellularity itself. The pre-animal world was a world of single-celled predators and prey, and one option for those wanting to avoid ending up as prey is to become too big to comfortably engulf. Then when cells have come together to make animals, action has to be reinvented at the larger scale. New kinds of coordination are needed. The fitful sponges are on the edge of this rediscovery, a partial case. In cnidarians, action exists again unambiguously, with motion and rearrangements on the grand scale of the animal body.
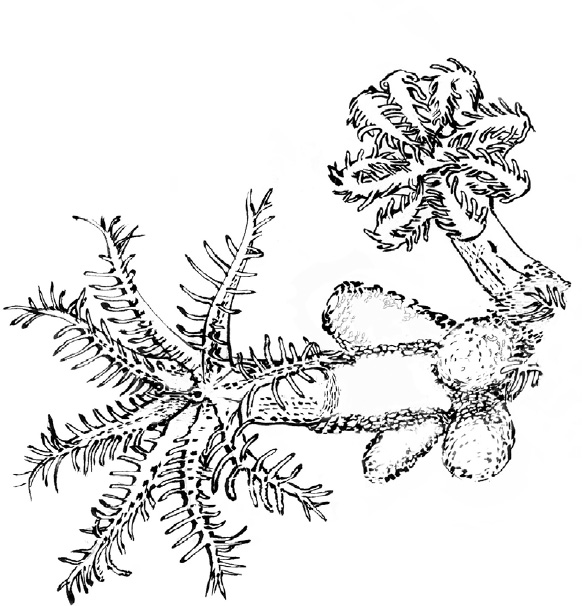
Those reaches and clenchings are not all that cnidarians can do. Another important capacity, an old action of a different kind, is the firing of their stinging cells, nematocytes. All or just about all cnidarians have stinging cells. Some of the stings, especially on anemones, are so feeble they are hardly noticed by a person. Others, on box jellyfish, can kill us outright. The stingers vary but are similar enough in form that they probably came from a single invention early in the cnidarian part of the tree, then passed down many branches.
In the dramatic and sometimes dangerous cases, a harpoon is coiled inside a cell. The cells with harpoons are surrounded by sensing cells and other controllers in a “battery” (this seems to be an artillery metaphor, and an apt one). The harpoon, when triggered, reaches extraordinary accelerations and high speeds over a tiny distance. In a harpoon release, the behavior itself—the motion executed—is performed by a single cell. That cell has helpers around it, sensors and the like, but no coordination between cells is needed for the act itself. Compare this to the soft coral’s reach. This is not the action of a cell, but a combination of countless contractions by different cells, motions that have to occur together in a concerted way. What I am highlighting here, marking as a special invention, is action that involves coordination across vast scales from a cell’s point of view. The origin of this is what we find echoed in the soft coral’s reach.
Even if these cnidarian behaviors are echoes of early animal actions, why do I choose this one? What about the coordinated swimming of a medusa? The medusa stage is often seen as a later addition to the cnidarian way of life, with the polyp stage evolving earlier. But a more important point is that if you look at a jellyfish swimming and a soft coral reaching, these are in a sense the same motions. Whether swimming or grasping, in a bell or a cup, both motions involve contraction around a radial form. A polyp and medusa can look very different, but in basic layout, a medusa is a polyp upside down. In the medusa, a radial contraction results in a swimming motion; in a polyp, the animal is stationary and the result is a grasp.
If we are looking for the “first actions,” another question is why we should focus on movement, as opposed to the other main thing living organisms do, which is making chemicals. Moving parts of your body and brewing chemicals are both ways of achieving effects that help you make your way. That is true, but the advent of controlled motion on the scale of the animal body was still a landmark. Though action was not invented from scratch by cnidarians, we see in these animals action of a different kind and on a different scale. The bodies that enable these actions were a new kind of object in the world, and a new factor in making things happen.
The Animal Path
The outgrowth of the tree of life that became the animals fairly quickly accumulated a suite of innovations. Perhaps the most important was the nervous system.
Of the animals we have looked at so far, nervous systems are present in cnidarians and comb jellies, but not in sponges or placozoans. Nervous systems evolved early, perhaps just once, perhaps a couple of times. The basis for what nervous systems do is a pair of features that were present long before animals. These are the electrical “excitability” of cells—the capacity for a rapid change in electrical properties, discussed in Chapter 2—and chemical signaling between one cell and another. Nervous systems put those two ancient capacities together. When a cell excites—a sudden shift in its electrical properties—this event is usually confined to that one cell. It is restricted by the boundaries that mark off the cell as a unit. But one thing such a spasm can do is trigger the release of a chemical at the cell’s boundary, a chemical that can be picked up by another nearby cell. This in turn may make the second cell more (or less) likely to go through its own electrical changes. Chemical signaling together with excitability are central to how a nervous system works.
Nervous systems are full of cells that specialize in these sorts of interactions. These cells take a tree-like shape, featuring fine projections that bring one cell into chemical contact with a selected group of others. Nervous systems are usually said to be found only in animals (and most, not all animals), though cells with a combination of excitability and chemical signaling are found in other organisms as well. What makes nervous systems in the full animal sense special is those cells with the branching shape: neurons. These are found nowhere outside of animals. Having such cells changes the way influence works within a body. They enable fast and targeted interactions, in contrast to more diffuse patterns of influence in which a cell sends chemicals out to whoever is listening. A nervous system ties the body together in new ways. Lars Chittka, a biologist who works on bees, has an effective way of illustrating their power. A bee, he says, has a brain that is a cubic millimeter in size. That seems tiny. But, he adds, a single bee neuron can have the complexity of a full-grown oak tree, with respect to its branching. Each neuron can connect to ten thousand others.
The nervous system is a reworking of capacities seen over much of life, but in animals these capacities have been extended and made vastly more powerful. A useful reminder of how much these systems do for us is seen in the fact that “nerve toxins” are the main kind of fast-acting toxin, both in animals like snakes and in human misdeeds—notorious weapons like sarin, VX, and Novichok are nerve toxins. On first hearing about nerve toxins when I was young, I thought: It prevents you from feeling anything? You go numb? You can’t think? But nerve toxins shut down more than that. Death occurs often by asphyxiation or cardiac arrest. Our vulnerability to these chemicals—which are not intrinsically destructive, which do not lay waste to tissue, but instead interfere with tiny sites of interaction between cells—is indicative of how a nervous system ties the animal body together. A way to kill that body is by going after the messengers, hence going after the coordination of parts.
Another feature closely associated with the nervous system in evolutionary terms is muscle. A cnidarian’s actions, unlike the fainter motions of a sponge, are based in muscle. The previous chapter noted the invention of the cytoskeleton, an internal skeleton of mobile filaments inside some single-celled organisms. In animals, the coordination of those internal skeletons across many connected cells generates the innovation of muscle. It enables the coordinated contraction, and relaxation, of massive sheets of cells.