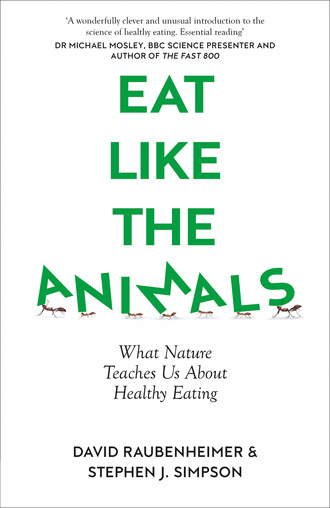
Полная версия
Eat Like the Animals
The scientific study of appetite is more recent. It all started with an important question: Exactly what is it in our bodies that makes us feel hungry? An early theory, which dates to 1912, was what has come to be known as the rumble theory. According to this, stomach fullness is the switch for appetite: hunger is turned on when the walls of an empty, churning stomach rub against each other (“rumble”) and turned off when it is full. The rumble theory took a fatal blow when it was later found that a stomach was not needed to feel hungry: patients who had had their stomachs surgically removed to treat cancer or ulcers continued to experience those familiar pangs.
Several theories followed, each one proposing a different measurement made by the body to tell our brains when we should eat. The thermostatic hypothesis suggested that animals ate to get enough energy to keep warm and stopped eating to prevent overheating; the glucostatic hypothesis identified blood sugar as the important link; the lipostatic theory implicated body fat reserves; and the aminostatic theory argued that amino acids circulating in the blood were responsible. While obviously different, all of these theories had in common that they identified a single component of the diet as the link between appetite and what the body needed: energy, sugars, fats, or amino acids.
In the 1930s, working quietly and inconspicuously with rats in his lab at Johns Hopkins University, was a young scientist named Curt Richter. The son of a German engineer, Richter had less time for theories than for performing clever experiments to measure exactly how the body instructs the brain to perform specific behaviors, and eating was no exception. Over a period of seventy years, working in the same laboratory, Richter made many discoveries that form an important backdrop to our research and the story in this book.
In one experiment, Richter operated on rats to trick their physiology into losing salt from the body at a fatal rate. And yet they didn’t die but rather just ate more salt to compensate for the increased losses. Importantly, they didn’t eat more food overall or more of other nutrients, only salt. He did similar experiments for calcium with the same result: the rats saved their own lives by eating more calcium but no more of other nutrients.
To ensure that what he was seeing was relevant to the normal lives of rats, Richter also studied their pattern of food selection in times when female rats naturally had an increased need for sodium and calcium, during pregnancy and lactation. As he suspected, here, too, they selected diets that were higher than usual in these nutrients. Richter’s experiments had shown that rats have not one appetite but at least two for different nutrients. All those other theories—thermostatic, glucostatic, lipostatic, and aminostatic—needed a rethink. Not because they were all wrong, but perhaps there were elements of truth in each.
This is where our own research on locusts entered the story. We proved that even in insects there are multiple appetites and that these can be used to select a balanced diet.
But appetites don’t exist only to tell us to start eating. Equally important, they also tell us when to stop. This involves nutrients in the food being released by digestion, getting absorbed into the bloodstream, and sending signals of fullness back to the brain. The only flaw is that these signals take time to kick in (in fact, some don’t act until after the end of a meal). The risk is that you will have overeaten by the time you get the message to stop. We all know the sensation of eating so much, so quickly, that we didn’t realize we were full ten minutes ago. In the meantime, we have delivered a calorie bomb to our system.
How can this be avoided? We need something to slow down our eating, fill us up quickly, and allow time for the slowly absorbed nutrients to reach our blood and announce to the brain that they are there. Luckily, nature has provided us that, too, in the form of a gut-stretcher that induces the feeling of fullness and slows the rate of gut emptying.
Fiber.
For herbivores like locusts and omnivores like us, fiber is the major source of bulk in plant foods. It forms the structure of plant cells and tissues and, as we explained in Chapter 2 and More on Nutrients, much of it is based on complex forms of carbohydrate that we are unable to digest using our own digestive enzymes. Some of that fiber, however, is digested by microbes that live in our guts—our microbiome, as those trillions of bacteria are known collectively.
In return, these microbes produce key nutrients (short-chain fatty acids, vitamins, and amino acids) that our bodies need. They also support our immune systems, keeping our intestines healthy, and even benefit our mental health. In addition to all that, gut microbes produce signals that make us feel full. They are an important part of our appetite control system.
Thanks to locusts and some geometry, we had glimpsed the beauty and power of nutrient appetites. We had seen how they worked together in perfect rhythm when possible—when the correct range of foods was available—to help animals solve the complex challenges of eating a balanced diet. We had also seen, in the locust experiment, that when things got rough and it wasn’t possible to eat a balanced diet, the appetites came into conflict. In that case, for locusts, the protein appetite took the lead, and carbs followed more passively.
We began to wonder whether locusts were just an elegant oddity that was not generalizable to the rest of the animal kingdom—let alone to humans. Or were we looking at something like a general rule of nature? If so, that could be important.
CHAPTER 4 AT A GLANCE
1 Animals have evolved separate appetites for protein, carbs, fat, sodium, and calcium. Together, the Big Five can signal a nutritionally balanced diet.
2 Fiber acts as a brake on appetite, preventing overeating and feeding the gut microbiome.
3 When offered an appropriate choice of foods, the protein and carbohydrate appetites work in harmony to guide locusts to consume a balanced diet.
4 But is nutrient balancing universal among all animals?
5
Seeking Exceptions to the Rule
WHEN SCIENTISTS ARE IN PURSUIT OF something potentially important, we are trained to temper our enthusiasms and continually ask: Could we be wrong? For us, that question took this form: Could it be that the power of multiple appetites to serve an organism’s nutritional needs was the exception rather than the rule?
Specifically, in our case, the question was whether the balancing act performed by the feeding locusts in our lab was the rule for other animals, and not just in laboratories but in natural environments. Nutrient balancing using multiple appetites was probably commonplace, we suspected.
This wasn’t merely wishful thinking by a pair of scientists wanting to have discovered something important. We had grounds to believe we might be right. In fact, we reasoned that what we were seeing was more or less a requirement for all living things.
A compelling reason for our belief comes first from the logic of Darwin. The mechanism by which organisms acquire their various features and skills involves a simple numbers game. Any characteristic that aids successful reproduction, provided it is at least partly heritable, will be passed on to the next generation—more so than characteristics that are unhelpful. Because these traits can be inherited, the offspring whose parents had the useful characteristic will themselves be more successful at reproduction, and in this way the helpful trait will eventually become more common and displace its less helpful equivalents in the population.
In terms of our research, everything we knew about biology was pointing us in the same direction: animals that balance their diets would be better equipped to reproduce than those that did not. For those unfortunate ones, eating would be like a lottery, leaving chance to decide which nutrient needs would be met and which would go hungry. Without appetites to guide the way, animals might get lucky some days and eat the mix of nutrients they need, but most of the time they would fail.
But this merely suggested what was likely, not necessarily what was true. How could we test it? The surest way would be to examine every species on the planet. But with the immense amount of work involved in our locust experiments still fresh in mind, we knew this was never going to happen. We hold the record for the number of different species whose nutritional wisdom has been studied (more than fifty by our reckoning—varying in size from ants to moose), and you will read many of their stories in this book. But that simply scratches the surface.
We needed a different approach.
The most effective way to learn whether nutrient balancing is the rule among animals or is restricted to a few, we realized, would be to reverse the question. We’d test the species that seemed to be least likely to balance their nutrient intake. If we were wrong, they’d show us. This kind of self-skepticism, in which theories are tested by seeing how much of a battering they can take and remain standing, is not only usual practice in science but a defining characteristic. This is what makes science science.
Anyway, now we needed to seek species that didn’t engage in nutrient balancing. If even the unlikeliest candidates were found to do so, we could be confident that most if not all do. Which species were particularly likely to disprove our theory? In a sense, we already had studied one of them. Of all animals, locusts were among the most renowned for an indiscriminate greed, devouring everything in their path. Having shown that even they meticulously compiled a precise mix of nutrients in their diet gave us confidence that so, too, would other species, especially those already known to be fussy eaters.
An even stiffer challenge then arose through a chance encounter involving a cacophony of chirping chickens, a student with a philosophical bent, and two halves of bugger-all.
It was 1997, when David was in the Lancaster lab of Oxford Zoology Department teaching a practical course in animal behavior. The students were busy with an experiment involving groups of recently hatched chickens. David was at the front of the class talking to the celebrated evolutionary biologist and devout atheist Richard Dawkins, who had stopped in to see what all the chirping was about.
Seeing his opportunity, an earnest young student named Stephen Jones approached and addressed Richard in a well-spoken English voice: “I am interested in writing an essay on postmodernist science. Would you be prepared to supervise this?”
“What is postmodernist science, in any case?” Richard asked in his characteristic laconic style. Then he immediately answered his own question: “Exactly two halves of bugger-all,” a pungent Britishism we can translate as “fuck-all,” or, if we’re being decorous, absolutely nothing.
Richard well knew—better than most—that “postmodernist science” is a form of cultural relativism, a philosophical view according to which science is just another belief system equivalent to any other, with no monopoly on the truth. This, he knew, was wrong for reasons we discussed above: science’s self-skeptical nature is an efficient filter for eliminating incorrect theories and in this way, over time, separating fact from belief.
Richard declined to supervise Stephen’s essay. But David, having developed an interest in what was often a fine line that separates “belief” from “fact” in nutrition science, accepted.
Stephen did a fine job. Even though his paper didn’t make any game-changing contributions to the philosophy of what is and what is not true, it would indirectly make an important contribution to our search for the truth about feeding.
Through working on the essay, Stephen developed an interest in doing PhD research and wanted to study something of practical importance. He chose to work on cockroaches, those widespread pests with filthy habits, a bad smell, and a reputation for spreading disease. We immediately saw an opportunity: cockroaches were also perfect animals for testing whether nutrient balancing is general to animals.
Here’s why. These unlikable creatures are extremely wily, adaptable, and hardy. They can live in virtually all environments from tropical and temperate forests to salt marshes, deserts, and cities, enduring circumstances that would endanger most species. In cities, they are equally at home scavenging in garbage bins, drains, and sewers as in restaurants, pantries, and, given half a chance, your dinner plate, even moving among these venues in a single sitting. Underlying this flexibility is a remarkable capacity to survive on a very wide range of diets, including no diet at all—they can live for a month without eating or drinking and over one hundred days on water alone. A few very special tricks underpin this nutritional hardiness.
Inside the hindguts of cockroaches are thousands of tiny spines. Each houses millions of bacteria, which can digest sources of carbohydrate that to most animals would be useless. Cellulose, for example, the stuff of which wood, paper, cardboard, and cotton fabric are made, can be eaten by cockroaches and used for energy courtesy of their spine-living bacteria. Considering that cellulose is the most abundant organic compound on Earth and few other animals can use it for energy, this is a handy advantage; it means that for cockroaches there is almost never a shortage of carbohydrate.
It doesn’t end there. All animals need to get rid of nitrogen wastes that result from various metabolic processes involving protein synthesis and breakdown. In mammals, this is the main function of urine, whereas most insects, birds, and reptiles dump it as a white paste of uric acid. If cockroaches eat too much protein, they, too, excrete the excess as nitrogen wastes. But unlike other animals, they don’t excrete it all: some is stored as tiny crystals in specialized cells called urocytes, which are found in the insect equivalent of a liver, the fat body. Alongside the urocytes is another kind of fat body cell, called mycetocytes. These maintain captive populations of bacteria that are unable to live anywhere else in the world. Their job is to use the nitrogen stored in the urocytes as a raw material to make amino acids, which they release into the blood for use by the cockroach to make protein. Mycetocyte bacteria are, in effect, onboard nitrogen recycling plants.
With such flexible carbohydrate and protein processing abilities, we reasoned, cockroaches would not need to bother nearly as much as other animals about eating the precise levels of sugars, starch, and protein required by their tissues. This, we believed, is why cockroaches can live on such a wide range of diets and why they can survive in so many habitats.
It is also why we were excited at the opportunity to test whether they actually do balance their intake of nutrients. If an animal that apparently has no need to eat precisely the right amounts of carbs and protein nonetheless does so, surely then other species that have a greater need would do likewise.
Stephen performed a clever experiment to test this. In a first step, he maintained cockroaches on one of three diets for two days: one group got high protein and low carb, one was fed high carb and low protein, and the third got intermediate levels of both nutrients. In human terms, this would be roughly equivalent to eating either only fish, only rice, or only a mix of fish and rice combined—sushi. After this period, the cockroaches were offered a buffet containing all three diets, from which they could freely select whatever they preferred.
We were surprised by the results. When Stephen plotted the data points in our usual protein-carbohydrate intake graph, we immediately saw that the cockroaches not only balanced their intake of nutrients, but did so with a precision and determination that rivaled anything we’d seen to that point, even to now.
The graph showed that in the final, buffet phase, those cockroaches that had previously been on the sushi-like feed, with balanced levels of protein and carbohydrate, chose from all three foods to compose a diet similar to what they had been eating. The other two groups initially selected only the food with the nutrient they were previously denied: if they had been forced to eat only carbs, they now chose protein, and vice versa. They continued eating this way for 10 hours, after which both groups began to eat all three foods. By 48 hours of buffet, they had hit their target nutrient intakes. From that point on, all three groups ate an identical mix of protein and carbohydrate and continued that way until 120 hours later, when the experiment ended.
The message couldn’t be clearer. Each insect had eaten the precise amounts of the nutrients needed to correct the imbalance we imposed on it, and once that had been achieved, they all selected an identical diet—which maintained the balanced nutritional state. The term nutritional wisdom was at the time in vogue. Here we had seen nutritional genius. The cockroaches had behaved like nutrient-seeking missiles.
Shortly after, Stephen left science to pursue a career in the church. We have yet to tell Richard Dawkins.
Thanks to the cockroaches, we had become more confident that nutrient balancing was not an arcane ability confined to a few species. But these intriguing if unpleasant creatures were of interest mainly to researchers in pest control. To extend our test of the generality of nutrient balancing in nature, we next turned to species that were widely believed not to balance their nutrient intake.
The perfect choice was predators. According to foraging theory, these animals would have no need to feed selectively to balance their nutrient intake because the foods of predators—the bodies of other animals—were thought to contain the same mix of nutrients as the animal eating them (making the axiom “You are what you eat” the literal truth). As a result, scientists believed, it should be effortless for predatory animals to consume a properly balanced diet, while we creatures who eat more than one thing would have to work harder at it.
If this were true, it had obvious implications for our theory of nutrient balancing: it would fail to apply to the very large group of animals that obtain their nutrition from eating other animals.
We needed to test this. A perfect opportunity arose when David examined the PhD thesis of a young Danish researcher, David Mayntz, on spiders.
That was an interesting experience. David’s own PhD defense, at Oxford University, was a serious affair, involving five hours of earnest discussion and argument with a small expert panel of examiners. Only after that was a decision made about whether the candidate had passed. If this is likened (with some justification) to a no-holds-barred kickboxing match, then a Danish PhD defense is more like the charade of professional wrestling. The candidate sits with the examiners at the front of a room, in this case on a stage, before a public audience usually including family and friends. By this point, it has already been decided that the thesis has passed, and the main purpose of the questioning is entertainment: it gives the candidate an opportunity to demonstrate their expertise and prowess in debating their research topic.
With his recently earned PhD degree, David Mayntz moved to Oxford to work with us applying Nutritional Geometry to predators. We designed an experiment like the one Stephen Jones had done on cockroaches. In a stroke of genius, David Mayntz suggested that we include in the experiment not just one but three species of predator, each differing in their hunting strategies. This presented a very stern test of our theory.
The first species, a ground beetle, moves around its environment searching for prey in much the same way that cockroaches forage. In the wild, these animals, theoretically at least, could select among the prey that they capture and eat.
The second was a wolf spider. Like the beetle, this species is mobile; but rather than searching for prey, it sits and waits for dinner to come to it.
The third species was least mobile of all: a web-building spider, which invests in building a trap for its prey.
We reasoned that if predators did balance their nutrient intake, the mobile beetle, which has opportunity to encounter many different prey types, would be the most likely to do so. The web-building spider, which has little choice over what gets trapped in its web, would be least likely to do so. The sit-and-wait predator would be somewhere in between because, even though it has little influence over which prey come within striking range, it can easily move among hunting spots to influence its opportunities.
We tested each species in an experiment that matched its ecology. We offered the mobile beetles a buffet-style test, like the cockroach experiment, in which the different test foods were available together. In the wild, web-building spiders have to make do with whatever happens to wander into their trap. So, rather than offer them a choice of prey in our experiment, we gave each a single victim that was either high or low in the nutrient they were lacking—fat or protein—and measured how the spiders responded to each. Like web builders, in nature, sit-and-wait predators can choose where they lay their ambush but not which prey come within striking distance. They, too, were tested on a single prey that was either high or low in the nutrient they lacked.
(How, you might wonder, did we get prey that differed in the balance of fat and protein they contained? The answer is that we raised the prey—flies—in our lab and gave them different diets. We designed some diets to make flies fat, and these chubby insects provided fatty meals for their predators, while other flies grew lean on low-fat, high-protein feed.)
The roving beetles behaved much like the cockroaches: if they had previously been restricted to low-fat prey, they specifically selected high-fat foods, and if previously restricted to low protein, they chose high protein. The sit-and-wait predator chose its nutrients by eating different amounts of the prey it had been offered, depending on its composition: the spider ate more of fatty animals if fat was needed and more of lean ones when protein was needed.
The web-building spider did the most remarkable thing of all. Its way of feeding is to inject into prey a cocktail of digestive enzymes, then suck out a soup of predigested nutrients from the body, discarding the remaining solids. We found that the discarded part of the body was particularly depleted in the nutrient most needed by the predator, suggesting that they could tailor the cocktail of enzymes they injected into prey in order to meet specific nutrient needs.
David Mayntz’s experiment had shown not only that all three predators balanced their nutrient intake but also that the mechanism for this differed with different foraging strategies. Some selected among prey, some regulated the amount of a specific prey they ate, and others selectively extracted nutrients from whatever prey was captured. It was looking increasingly unlikely that we would find a significant number of animal species that didn’t balance their nutrient intake.
Usually, when people think about predators, their thoughts are not on invertebrates like beetles and spiders but on charismatic examples like lions, tigers, and sharks. Did they, too, carefully mix foods in the exact proportions to balance their nutrient intakes? It seems ridiculous to consider a lion or shark sizing up its prey for body composition, but then in recent years our research had presented a series of surprises.
Even more ridiculous was the thought that we could do locust-like lab experiments on man-eaters. Happily, many of us share our houses with predators that are somewhat less likely to eat their experimenter.
An opportunity arose when we were contacted by Adrian Hewson-Hughes, a research scientist at a prominent pet food company. Adrian had come across our work and wondered whether it applied to domestic cats and dogs. It was the practical implications that interested Adrian and us, too, but we also saw a wonderful opportunity to answer the question of whether vertebrate predators balance their diets.