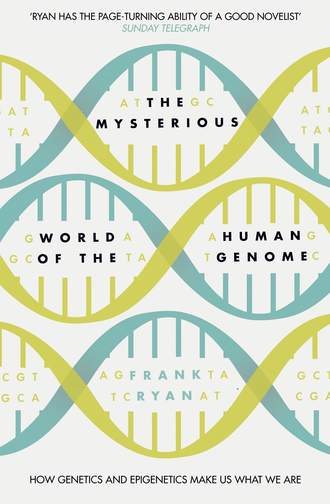
Полная версия
The Mysterious World of the Human Genome

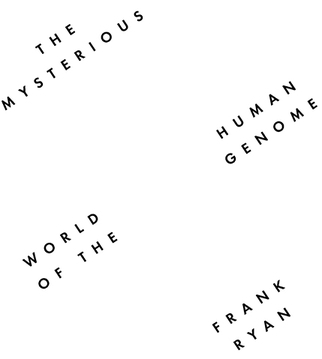
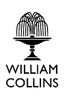
Copyright
William Collins
An imprint of HarperCollinsPublishers
1 London Bridge Street
London SE1 9GF
WilliamCollinsBooks.com
This eBook first published in Great Britain by William Collins in 2015.
Text © FPR-Books Ltd 2015
Diagrams by Mark Salwowski
Frank Ryan asserts his moral right to be identified as the author of this work.
A catalogue record for this book is available from the British Library.
Cover design by Jo Walker
Cover design © HarperCollinsPublishers 2015
All rights reserved under International and Pan-American Copyright Conventions. By payment of the required fees, you have been granted the non-exclusive, non-transferable right to access and read the text of this e-book on-screen. No part of this text may be reproduced, transmitted, down-loaded, decompiled, reverse engineered, or stored in or introduced into any information storage and retrieval system, in any form or by any means, whether electronic or mechanical, now known or hereinafter invented, without the express written permission of HarperCollins.
Source ISBN: 9780007549061
eBook Edition © June 2015 ISBN: 9780007549078
Version: 2016-03-02
Dedication
To Oswald T. Avery
Possibly I am a scientist because I was curious when I was young. I can remember being ten, eleven, twelve years old and asking, ‘Now why is that? Why do I see such a peculiar phenomenon? I would like to understand that.’
LINUS PAULING
Table of Contents
Cover
Title Page
Copyright
Dedication
Epigraph
Introduction
Chapter One: Who Could Have Guessed It?
Chapter Two: Dna Is Confirmed as the Code
Chapter Three: The Story in the Picture
Chapter Four: A Couple of Misfits
Chapter Five: The Secret of Life
Chapter Six: The Sister Molecule
Chapter Seven: The Logical Next Step
Chapter Eight: First Draft of the Human Genome
Chapter Nine: How Heredity Changes
Chapter Ten: The Advantage of Living Together
Chapter Eleven: The Viruses That Are Part of Us
Chapter Twelve: Genomic Level Evolution
Chapter Thirteen: The Master Controllers
Chapter Fourteen: Our History Preserved in Our Dna
Chapter Fifteen: Our More Distant Ancestors
Chapter Sixteen: The Great Wilderness of Prehistory
Chapter Seventeen: Our Human Relatives
Chapter Eighteen: The Fate of the Neanderthals
Chapter Nineteen: What Makes You Unique
Chapter Twenty: The Fifth Element
Bibliography
Chapter Notes
Index
Acknowledgements
By the Same Author
About the Publisher
Introduction
No special act of creation, no spark of life was needed to turn dead matter into living things. The same atoms compose them both, arranged only in a different architecture.
JACOB BRONOWSKI, THE IDENTITY OF MAN
Bronowski begins his more famous book, The Ascent of Man, with the words, ‘Man is a singular creature. He has a set of gifts which make him unique among the animals: so that, unlike them, he is not a figure in the landscape – he is a shaper of the landscape.’ But why should we humans have become shapers of the landscape rather than mere figures inhabiting it? We differ from, say, a sea horse or a cheetah because our genetic inheritance, the sum of the DNA that codes for us, is different in humans when compared to the horse or the cheetah. We call this the genome, or, to be more specific in our case, the human genome.
Our genome defines us at the most profound level. That same genome is present in every one of the approximately 100,000 billion cells that make us who we are as individual members of the human species. But it runs deeper than that. In more personal terms, in myriad tiny variations that we each possess and are individual to us only, it is the very essence of us, all that, in genetic and hereditary terms, we have to contribute to our offspring, and through them to the sum total evolutionary inheritance of our species. To understand it is to know, in the most intimate sense, what it means to be human. No two people in the world today have exactly the same genome. Even identical twins, who will have been conceived with exactly the same genome, will have developed tiny differences between their genomes by the time of their births: differences that may have arisen in parts of their genomes that don’t actually code for what we normally mean by genes.
How strange to realise that there is actually more to our individual genome than genes alone. But let us put aside such details for the moment to focus on the more general theme. How could a relatively simple chemical code give rise to the complexity of a human being? How could our human genome have evolved? How does it actually work? Immediately we are confronted by mysteries.
To answer these questions we need to explore the genome’s basic structure, its operating systems and its mechanisms of expression and control. Some readers might react with incredulity. Surely any such exploration promises a journey into extraordinary complexity, one that is far too obscure and scientific for a non-scientist reader? In fact this book is aimed at exactly such a reader. As we shall see, the basic facts are easy enough to grasp, and the way to grasp them is to break the exploration into a series of simple, and eminently logical, stages. This journey will lead us through a sequence of remarkable revelations about our human history – even into the very distant past of our ancestors’ lives and their prehistoric exploration of our beautiful life-giving planet.
The exploration will raise other, equally important, questions, too. How, for example, does this extraordinary entity that we call the human genome enable our human reproduction – the fertilisation of our maternal egg with the paternal sperm? How does it control the quasi-miracle of the developing embryo within the mother’s womb? Returning to generalities for the moment, though, we can be sure that an important ingredient of the genome, and its essential nature, is memory – the memory, for example, of the totality of every individual human’s genetic inheritance. But how exactly does it perform this remarkable feat of memory? We know already that this wonder chemical we call DNA works like a code, but how could any code recall the complex instructions that go into the making of cells and tissues and organs, and then once made, bring them into function in the single coordinated whole that comprises the human being? Even then we have hardly begun to confront the mysteries of the human genome. How does this same extraordinary structure acquire the programming that gifts the growing child with the wonder of speech, that bestows the related capacity for learning, writing and education, and which makes possible the maturing of the newborn to the future adult, who then repeats the cycle all over again when he or she becomes a parent in turn?
The wonder is that all of this might be encompassed in a minuscule cluster of chemicals including, but not exclusive to, the master molecule we call deoxyribonucleic acid – or DNA. This chemical code somehow records the genetic instructions for making us. Built into that code must be the potential for individual liberty of thought and inventiveness, enabling every human artistic, mathematical and scientific creativity. It gives rise to what each of us thinks innately as our private inviolate ‘self’. Somehow that same construction of ‘self’ made possible the genius of Mozart, Picasso, Newton and Einstein. It is little wonder that we look at the repository of such potential with awe. No more is it surprising that we should want to understand this mystery that lies at the very core of our being.
Only recently have we come to understand the human genome in sufficient depth and subtlety to be able to put together its marvellous story – to discover, for example, that there is rather more to it than DNA alone. This is the story I shall attempt to convey in this book.
A few years ago I gave a lecture on a related theme at King’s College London. The chairman asked me if I planned to write a book about it. When I said yes, he asked me to please write it in words that a lay reader, like himself, could readily understand.
‘Just how simple do you want me to make it?’
‘I want you to assume that I – your reader – know nothing at all to begin with.’
This, then, I promise to do. There will be no complicated scientific language, no mathematical or chemical formulae or unexplained jargon, and I shall introduce no more than a handful of simple illustrations. Instead I shall begin from first principles and assume that the readers of this book know little about biology or genetics. Even non-biologists might recall the many surprises when the first rough draft of the human genome was announced to the world, in 2001.The discoveries since then have confirmed that a good deal of the human genome – in its evolution, structure and workings – is far from what we had earlier imagined. Those surprises do not diminish the importance of the wealth of knowledge that had gone before, but rather, like all great scientific discoveries, they enhance it. Thanks to this new understanding, humanity has entered what I believe to be a golden age of genetic and genomic enlightenment, which is already being extrapolated to many important fields, from medicine to our human prehistory. I think society at large deserves to understand this and what it promises for the future.
one
Who Could Have Guessed It?
The large and important and very much discussed question is: How can the events in space and time which take place within the spatial boundary of a living organism be accounted for by physics and chemistry?
ERWIN SCHRÖDINGER
In April 1927 a young Frenchman, René Jules Dubos, arrived at the Rockefeller Institute for Medical Research, in New York, on what would appear to have been a hopeless mission. Tall, bespectacled and a recent graduate of Rutgers University, New Jersey, with a PhD in soil microbiology, Dubos had an unusually philosophical attitude to science. He had become convinced, through the work of eminent Russian soil microbiologist Sergei Winogradsky, that it was a waste of time studying bacteria in test tubes and laboratory cultures. Dubos believed that if we really wanted to understand bacteria we should go out and study them where they actually lived and interacted with one another and with life in general, in the fields and the woods – in nature.
On graduation from Rutgers Dubos had found himself unemployed. He had applied to the National Research Council Fellowship for a research grant but had been turned down because he wasn’t American, but somebody had scribbled a handwritten message on the margin of the rejection letter. Dubos would later reflect upon the fact that it was written in a female hand, almost certainly added as a kindly afterthought by the official’s secretary. ‘Why don’t you go and ask advice and help from your famous fellow countryman, Dr Alexis Carrel, at the Rockefeller Institute?’ Dubos duly wrote to Carrel, which brought him, in April 1927, to the building on York Avenue, on the bank of the East River.
Dubos knew nothing about Carrel, or indeed about the Rockefeller Institute for Medical Research, and was surprised on his arrival to discover that Carrel was a vascular surgeon. Dubos had no academic knowledge of medicine and Carrel knew nothing about the microbes that lived in soil. The outcome of their conversation was all too predictable; Carrel was unable to help the youthful microbiologist. Their conversation closed about lunchtime and Carrel did Dubos the courtesy of inviting him to have lunch with him in the Institute’s dining room, which had the attraction for a hungry Frenchman that they served freshly baked bread.
It seemed entirely by accident that Dubos found himself sitting at a table next to a small, slightly built gentleman with a domed bald head who addressed him politely in a Canadian accent. The gentleman’s name was Oswald Theodore Avery. Although Dubos later confessed that he knew as little about Avery as he did of Carrel, Professor Avery (his close associates referred to him as ‘Fess’) was eminent in his field, which was medical microbiology. It would prove to be a meeting of historic importance both to biology and to medicine.
Avery subsequently hired Dubos as a research assistant, in which role Dubos discovered the first soil-derived antibiotics. Meanwhile Avery led his small team – who were working on what he called his ‘little kitchen chemistry’ – on another quite different quest, one that would help unravel the key to heredity. Why then does society know little to nothing about this visionary scientist? To understand how this anomaly came about we need to go back in time to the man himself and the problems he faced three-quarters of a century ago.
*
In 1927, when Dubos first met Avery, the principles of heredity were poorly understood. The term ‘gene’ had been introduced into the nomenclature two decades earlier by a Danish geneticist, Wilhelm Johannsen. Curiously, Johannsen had adopted a vague concept of heredity, known as ‘pangene’, that was first proposed by Charles Darwin himself. Johannsen modified it to take on board the belated discovery of the pioneering work of Gregor Mendel, which dated back to the nineteenth century.
Readers may be familiar with the story of Mendel – the cigar-smoking, Friar-Tuck-like abbot of an Augustinian monastery in Brünn, Moravia (now the Czech Republic) – who undertook some brilliantly original studies of the peas he cross-bred in the monastery vegetable garden. From these studies Mendel discovered the basis of what we now know as the laws of heredity. He found that certain characteristics of the peas were transmitted to the offspring in a predictable manner. These characteristics included tallness or dwarfishness, presence or absence of the colours yellow or green in the blossoms or axils of the leaves, and the wrinkled or smooth skin in the peas. Mendel’s breakthrough was to realise that heredity was stored within the germ cells of plants – and this would subsequently be extrapolated to all living organisms – in the form of discrete packets of information that somehow coded for specific physical characters or ‘traits’. Johannsen coined the term ‘gene’ for Mendel’s packet of hereditary information. At much the same time, a combative British researcher, William Bateson, extrapolated the term ‘gene’ to the discipline he now called ‘genetics’ to cover the study of the nature and workings of heredity.
Today, if we visit the free dictionary online, we get the following definition of a gene: ‘The basic physical unit of heredity; a linear sequence of nucleotides along a segment of DNA that provides the coded instructions for the synthesis of RNA, which, when translated into protein, leads to the expression of hereditary character.’ But Mendel had no notion of genes as such, and he certainly knew nothing about DNA. His discoveries languished in some little-read papers for forty years before they were rediscovered and their importance was more fully understood. But in time his idea about the discrete packets of heredity we now call genes helped to answer a very important medical mystery: how certain diseases come about through an aberration of heredity.
We now know that genes are the basic building blocks of heredity in much the same way that atoms are the physical units that make up the physical world. But during the early decades of the twentieth century, nobody had any real notion of what genes were made from, or how they worked. But here and there, people began to study genes in more depth by examining their physical expression during the formation of embryos or in the causation of hereditary diseases. Fruit flies became the experimental model for pioneering research in the laboratory of Chicago-based geneticist Thomas Hunt Morgan, where researchers located genes, one by one, onto structures called chromosomes, which were themselves located within the nuclei of the insect’s germ cells. The botanical geneticist Barbara McClintock confirmed that this was also the case for plants. McClintock developed techniques that allowed biologists to visualise the actual chromosomes in maize, leading to the groundbreaking discovery that, during the formation of the male and female germ cells, the matching, or ‘homologous’, chromosomes from the two parents lined up opposite each other and then the chromosomes swapped similar bits so that the offspring inherited a jumbled-up mixture of the inheritance from the two parents. This curious genetic behaviour (which is known as ‘homologous sexual recombination’) is the explanation of why siblings are different from one another.
By the early 1930s biologists and medical researchers knew that genes were actual physical entities – chemical blocks of information that were lined up like beads in a necklace along the lengths of chromosomes. In other words, the genome could be loosely compared to a library of chemical information in which the books were the chromosomes. The discrete entities known as genes could then be compared to discrete words in the books. The libraries were housed in the nuclei of the germ cells – in human terms, the ova and sperm. Humans had a total stack of 46 books, which were the summed complement of ova and sperm, in every living cell. This came about because the germ cells – the ovum and the sperm – contained 23 chromosomes, so that when a human baby was conceived the two sets of the parental chromosomes united within the fertilised ovum, passing on the full complement of 46 chromosomes to the offspring. But this initial unravelling of the ‘heredity mystery’ merely opened up a Pandora’s box of new mysteries when it came to applying genetics to the huge diversity of life on our fecund planet.
For example, did every life form, from worms to eagles, from the protozoa that crawled about in the scum of ponds to humanity itself, carry the same kinds of genes in their nuclei-bound chromosomes?
The microscopic cellular life forms, including bacteria and archaea, do not store their heredity in a nucleus. These are called the ‘prokaryotes’, which means pre-nucleates. All other life forms store their heredity in nuclei and are known collectively as ‘eukaryotes’, which means true nucleates. From the growing discoveries in fruit flies, plants and medical sciences, it was becoming rather likely – excitingly so – that some profound commonalities might be found in all nucleated life forms. But did the same genetic concepts, such as genes, apply to the prokaryotes, which reproduced asexually by budding, without the need for germ cells? At this time within the world of early bacteriology there was even a debate as to whether bacteria should be seen as life forms at all. And viruses, which were for the most part several orders of magnitude smaller than bacteria, were little understood.
Over time many researchers came to see bacteria as living organisms, classifying them according to the binomial Linnaean system; so, for example, the tuberculosis germ was labelled Mycobacterium tuberculosis and the boil-causing coccoid germ was labelled Staphylococcus aureus. Oswald Avery, with his extremely conservative nature, kept his options open, eschewing the binomial system and referring to the TB germ as the ‘tubercle bacillus’. It is instructive for our story that Dubos, who came to know Avery better than any other colleague, would observe that ‘Fess’ was similarly conservative in his approach to laboratory research. Science must adhere with a puritanical stringency to what can be logically observed and definitively proven in the laboratory.
In 1882 German physician Robert Koch discovered that Mycobacterium tuberculosis was the cause of the greatest infectious killer in human history – tuberculosis. Koch constructed a code of logic that would be applied to bugs when first determining if they caused specific diseases. Known as ‘Koch’s postulates’, this was universally adhered to, and once a causative bug had been identified it was studied further under the microscope. Thus the bug was duly classified in a number of ways. If its cells were rounded in shape it was a ‘coccus’, if a sausage shape it was a ‘bacillus’, if a spiral shape it was a ‘spirochaete’. Bacteriologists methodically studied the sort of culture media in which a bug would grow best – whether in agar alone, or agar with added ox blood, and so on. They also studied the appearance of the bacterial colonies when they were grown in culture plates – their colours, the size of the colonies, whether they were rough in outline or round and smooth, raised or flat, stellate, granular or daisy-head. So the textbooks of bacteriology extended their knowledge base on a foundation of precise factual study and observation. And as understanding grew, this newfound knowledge was applied to the war against infection.
One of the useful things they learnt about disease-causing, or ‘pathogenic’, bacteria was that the behaviour of the disease, and thus of the bug itself in relation to its infected host, could be altered by various deliberate means: for example, through repeated cultures in the laboratory, or by repeatedly passing generations of the bug through a series of experimental animals. Through such manipulations it was possible to make the disease worse or less severe by making the bug either ‘more virulent’ or ‘attenuated’. Bacteriologists looked for ways to extrapolate this to medicine. In France, for example, the eminent Louis Pasteur used this principle of attenuation to develop the first vaccine to be used successfully against the otherwise universally fatal virus infection of rabies.
One fascinating observation that came out of these studies was the fact that, once a bug had been attenuated or been driven to greater virulence, the change in behaviour could be ‘passed on’ to future generations. Could it be that some factor of the bug’s own heredity had been altered to explain the change in behaviour?
Bacteriologists talked about ‘adaptation’, using the same term that was coming into vogue with evolutionary biologists when referring to evolutionary change in living organisms as they adapted to their ecology over time. While it was too early to be sure if bacterial heredity depended on genes, these scientists linked it to the physical appearance of bugs and colonies, or to the bugs’ internal chemistry, and even to their behaviour in relation to their hosts. These were measurable properties, the bacterial equivalents of what evolutionary biologists were calling the ‘phenotype’ – the physical make-up of an organism as opposed to what was determined by the hereditary make-up, or ‘genotype’.